Fig. 12.1
45 ° Fundus photograph of a 35 year old type 1 diabetic patient after 12 years of disease. No signs of diabetic retinopathy detectable
One disease that frequently strikes in the eye is diabetes , and diabetic retinopathy is a frequent encounter in daily medical care. The purpose of this chapter is to a. outline the clinical features of diabetic retinopathy, b. describe model systems which are used to assess the pathogenesis of diabetic retinopathy, and c. to align current knowledge about functional and structural abnormalities caused by factors or pathways discussed elsewhere in this book.
12.2 A Brief Epidemiology of Diabetic Retinopathy
Diabetic retinopathy is a frequent complication of diabetes of both, type 1 and type 2. The overall prevalence of any diabetic retinopathy worldwide is 34.6 %, and of sight-threatening diabetic retinopathy (either clinically significant macular edema (CSME) or proliferative diabetic retinopathy) is 10.2 % [1]. The highest prevalence rates is in African americans and the lowest is in asians. Retinopathy is more frequent in type 1 than in type 2 diabetes, and increases with disease duration, higher glucose/HbA1c levels, blood pressure, and cholesterol. Recent reanalysis of the landmark Wisconsing Epidemiology Study of Diabetic Retinopathy (WESDR) revealed a cumulative incidence of any retinopathy in the cohort surviving 25 years of 97 %, a cumulative rate of retinopathy progression of 83 %, and a rate of progression towards proliferative retinopathy of 43 %. These data confirm, that even after many years of implementation of novel therapies such as intensified insulin therapy, there is still an almost 100 % chance to develop some, and a 50 % chance of developing sight-threatening retinopathy requiring laser therapy. A recent survey of 8700 type 1 diabetic patients from a large data base in Germany and Austria demonstrated a cumulative proportion of any retinopathy of 84.1 %, after 40 years of diabetes, and a cumulative proportion of 50.2 % of advanced retinopathy [2] confirming the above numbers also apply to the local setting. However, advanced proliferative stages appear to decline to some extent in incidence [3]. The follow-up analysis of the Diabetes Control and Complication Trial/Epidemiology (DCCT/EDIC) suggests that the earlier normoglycemia is established, the better the retinal outcome is. Comparing the two treatment groups of DCCT after 30 years of disease, the cumulative incidence of PDR was 21 % in former intensive group, and 50 % in the former conventional group. The latter was comparable with the 47 % cumulative incidence of a population based group of similar diabetes duration. The implementation of strict glycemic control and other measures to normalize the metabolic profile in type 1 diabetes will further decrease the incidence of diabetic retinopathy. This and the translation of DRS and ETDRS findings, which demonstrated that timely laser treatment preserves vision, into treatment of advanced retinopathy stages may also affect visual prognosis in type 1 diabetes [4]. At present, it remains unclear whether the trend of declining retinopathy also applies to type 2 diabetes. On one hand, the numbers of persons with type 2 diabetes increases dramatically (rising the numbers of susceptible patients), on the other hand, implementation of metabolic control, polypharmacy, and timely treatment, in particular for CSME, reduces the numbers of patients with vision threatening retinopathy.
Diabetes is a systemic disease, affecting other organs, such as the kidney. Coincident nephropathy has a major impact on the progression of retinopathy to vision-threatening stages in both, type 1 and type 2 diabetes. Almost all patients with proliferative diabetic retinopathy have some degree of nephropathy. In the WESDR, the odds ratio for having retinopathy in microalbuminuric patients was 3.78 for the younger and 1.8 for the older group [5]. This association is confirmed in a recent study, in which the OR for severe retinopathy in microalbuminuric type 1 patients was 4.1 [95 % CI 3.4–4.9]. In patients with even more progressed renal damage as reflected by the transition to proteinuria, the increase in risk for sight-threatening retinopathy stages multiplies. In Danish type 1 diabetic patients, the 5 year cumulative incidence of PDR was 74 % in patients with gross proteinuria, but only 14 % in patients without proteinuria. The OR for advanced retinopathy in macroalbuminuric patients with type 1 diabetes in a large German study was 8.6 [95 % CI 6.4–11.5].
Patients with CSME may represent a special subgroup with possible shared pathogenesis resulting in a generalized vascular hyperpermeability. In a study of 40 type 2 diabetic patients, Knudsen et al. found a significant correlation of urinary albumin excretion and transcapillary albumin escape rates with diabetic macular edema as measured by optical coherence tomography [6]. Since generalized vascular permeability paralled vascular leakiness in target organs of diabetic injury, the conclusion was that with increasing albuminuria the impact on maculopathy would also increase. As a clinical correlate, a strong association of CSME with proteinuria in type 2 diabetic caucasion patients was found [7]. Together, these data suggest that diabetic retinopathy is part of a systemic disease in which the interaction of eye and kidney plays a particular role. This needs to be considered when diabetic retinopathy is used as a paradigm of a neovascular disease.
12.3 Risk Factors for Diabetic Retinopathy—the Impact of Glucose Revisited
Classical risk factors of diabetic retinopathy are disease duration, quality of glucose control, hypertension, lipids, gender, and hormonal changes. Chronic hyperglycemia is the single most important risk factor causing diabetic tissue damage, but its relative contribution has probably been overestimated since the DCCT and the UKPDS have reported beneficial effects of good glycemic control for the prevention of diabetic retinopathy [8, 9]. Analysis of the DCCT data revealed that improved control (i.e. lowering of HbA1c from 9 to 7 %) reduced sustained retinopathy progression by 73 %. However, in the analysis of the entire group, HbA1c and diabetes duration (i.e. the measure of glycemic exposure) explained only approximately 11 % of the variation in retinopathy risk [10]. From that, it was concluded that the remaining 89 % in the variation in risk is explained by factors related to glycemia, not reflected by HbA1c.
Moreover, using digitized photographs capturing the retinal vasculature in patients whose underlying risk profile differs fundamentally from diabetic patients, it was found that up to 12 % of “healthy” (i.e. non diabetic and non hypertensive patients) develop a retinal phenotype that matches mild stages of diabetic retinopathy. These data indicate that the retina is affected by mechanisms which are unrelated to glycemia, but that hyperglycemia still plays a role in the incipience and propagation of retinal damage.
12.4 Retinopathy as a Risk Indicator for Cardiovascular Disease
It is well documented that kidney disease is associated with excess cardiovascular morbidity and mortality in diabetes. The association between diabetic retinopathy and cardiovascular disease, however, had only been documented for advanced retinopathy stages, suggesting that only the subgroup of patients who developed proliferative diabetic retinopathy had an increase in cardiovascular mortality that was independent of classical CVD risk factors. In fact, the WESDR study was the first large study to demonstrate an excess mortality risk of death in patients with diabetic retinopathy, independent of important CVD risk factors such as age, diabetes duration, glycemia and gender [11]. Interestingly, the retinopathy-CVD association is less consistent in type 1 diabetes, mostly because of the role of age in CVD mortality. According to recent data, however, the risk association between retinopathy and CV mortality extends to any retinopathy which indicates that the development of any retinopathy in a person with diabetes is a biomarker for increased mortality. In this metaanalysis including over 19,000 patients from 20 studies, the OR for the composite outcome in type 2 diabetic patients, when adjusted for CV risk factors, was 1.61 (95 % CI 1.32–1.9) for any retinopathy, and 4.22 (95 % CI 2.81–6.33) for advanced retinopathy. For type 1 diabetes, the corresponding OR for any retinopathy was 4.10 (95 % CI 1.50-11.18), and 7.00 (95 % CI 2.22-20.0) for advanced retinopathy. When limiting the analysis to the studies that reported all cause mortality, the meta-analysis of the association between presence of retinopathy and all cause mortality was approximately 2.5 fold for both, type 1 and type 2 diabetes [12]. This is a strong indicator that the retinal vasculature is a mirror of general vascular risk.
12.5 Retinal Vascular Pathology in Diabetes
The diagnosis and staging of diabetic retinopathy is still based exclusively on signs of vascular dysfunction and structural damage. However, novel insights into the complex cellular interactions in the diabetic retina indicate that retinopathy is not only vascular disease , and that changes of the neural retina may even precede and predict vascular abnormalities (www.laskerfoundation.org/programs/irrf.htm). The implementation of sensitive diagnostic tools into research has opened the view on retinopathy as being the result of damage to multiple different cell types in the retina.
The common characteristics of diabetic vascular damage is increased vascular permeability and progressive vascular occlusion. Clinically, retinopathy is devided into two distinct stages, non-proliferative and proliferative diabetic retinopathy. Only late stages affect vision. Diabetic macular edema is defined as a condition resulting from progressive capillary dropout that causes thickening of the macula through accumulation of extracellular fluid, frequently accompanied by lipoprotein depositions and , if the fovea is involved, vision loss. Any of these advanced stages initiates with vascular changes which are readily detectable by fundus inspection. The earliest and most relevant sign of diabetic retinopathy is the microaneurysm (Fig. 12.2a and b). Clinically, it appears as a red dot of > 20 µm in diameter (Fig. 12.2a). Microaneurysms are often hypercellular, indicating angiogenic activity (Fig. 12.2b). Indeed, microaneurysms are typically located adjacent to areas of capillary dropout, suggesting hypoxic stimulation, and are spherical clusters of cells which stain for endothelial markers, while endothelial cells in capillaries of the vicinity remain mostly negative. This local proliferative response to vascular changes suggests an early abortive attempt of neovascularization amidst vasoregression. Later, microaneurysms can clinically disappear because intraluminar microthrombi occlude them. This can simulate clinical improvement. Both, microaneurysms and dot hemorrhages characterize mild non-proliferative diabetic retinopathy. Around capillaries and microaneurysms, lipoproteins can leak from capillaries as hard exsudates which are yellow, sharply bounded, and variable in size and form. When the macula is not affected, vision remains clinically unaltered. With progressive capillary dropout, avascular areas become confluent, and the retina responds differently to progressive ischemia. On the arteriolar site, microinfarcts of small arterioles disclose as “cotton-wool-spots”, representing nerve fiber swelling in the inner retina. On the venolar site, saccular bulges (“venous beading”) develop which are formed by hypercellular venolar segments. Subsequently, new vessel form within the level of the retina, or microaneurysms become numerous and leaky. This stage represents severe, (“preproliferative”) non-proliferative diabetic retinopathy at which the risk of blinding proliferative disease is high (Fig. 12.3). Proliferative diabetic retinopathy is defined by growth of new vessels into the vitreous, mainly originating from peripheral venules (NVE, neovascularization elsewhere) or from the optic disc (NVD, neovascularisation from the disc) (Fig. 12.4). Intravitreal hemorrhages cause acute vision loss, while the formation of fibrovascular tissue responding to the intravitreal discharge of inflammatory cells and its contraction is the cause of permanent vision loss through retinal detachment .

Fig. 12.2
panel a: Fundus photograph of a 48 year old type 1 diabetic patients after 23 years of disease. Arrows: microaneurysms/dot hemorrages defining mild non-proliferative diabetic retinopathy Panel b: Retinal digest preparation showing microaneurysms (arrows) in the vicinity of acellular capillaries. Original magnification 200x. Periodic acid Schiff stain

Fig. 12.3
Severe non-proliferative diabetic retinopathy showing venous beading (arrow upper right quadrant), intraretinal loop formation (arrow lower right panel), intraretinal microvascular abnormalities (IRMA) (arrows upper and lower left and at the optic disc)

Fig. 12.4
Fundus photograph showing neovascularization from the disc
Taken together, the clinical distinction between non-proliferative and proliferative diabetic retinopathy is based on clinical decision-making, not on the inherent underlying vascular processes. From a vascular biology point of view, even the initial clinical stages are proliferative in character, suggesting that the retina in diabetes responds with a continuum of proliferative vascular changes.
However, in a subset of patients, the tissue response is not angiogenic, but boosting permeability. When the macular is involved, retinal edema becomes particularly significant, and the resulting entity is diabetic macular edema, and clinically significant, if edema formation involves one disc diameter around the macular. When the edema affects the spatial integrity of the macular area, vision may become impaired. Clinically, macular edema is frequently accompagnied by hard exsudates which represent intraretinal extravasations of lipids at the border between edematous and nonedematous areas. They are considered hallmarks of focal breakdown of the blood retinal barrier, either widespread from large areas of affected capillaries or focal from microaneurysms. The parallel occurrence of leaky and non leaky microaneurysms, and the differences in patient characteristics suggests that local factors determine the clinical phenotype independent of common underlying pathogenetic abnormalities. Notably, the assocation between visual function as measured by acuity, and macular thickness as measured by optical coherence tomography (OCT) is not particularly strong which is of clinical importance when anti-edematous therapies are evaluated.
12.6 Animal Models—do they Reflect Clinical Retinopathy?
Animal models are widely used to study diabetic retinopathy. This is a. the consequence of the paucity of adequate human material, in particular of early stages, or b. the consequence of the availability of models with distinct genetic manipulations, in which the retinal phenotype is studied under diabetic conditions, or as a surrogate. Animal models are a crucial part to understand the pathogenesis, and to identify promising targets for treatment in humans.
Histopathological changes described in animal models of diabetic retinopathy may represent only preclinical human retinopathy, as outlined below, and may vary depending on the model. The only consistent changes that diabetic animals develop are the loss of pericytes and the degeneration of retinal capillaries (also termed vasoregression). Vasoregression and pericyte dropout also occur in the human diabetic retina speaking for the translational validity of animal models. Extensive reviews have highlighted the role, advantages and disadvantages, and other aspects of in vivo models of diabetic retinopathy [13]. In essence, there is no animal model that perfectly reflects human pathology (as there is no animal model of human diabetic nephropathy), and the need for such models has been clearly identified (see www.diamap.eu—5- microvascular complications). It must be emphasized that there is no diabetic animal that develops proliferative retinopathy comparable to humans. The reason for this observation is unclear, and short life span and differences in biochemical and cell biological factors have been cited as underlying causes. Thus, the rationale to study pathogenetic aspects in diabetic animals that develop only preclinical lesions is to identify mechanisms that are involved in the incipient changes in the diabetic retina such as pericyte loss and/or vasoregression for which human material is unavailable.
In chemically induced and spontaneous diabetic rats, pericyte dropout, acellular capillaries and basement membrane thickening consistently develop. Acellular capillaries become significantly more numerous after 6 months of diabetes , with considerable variability in different rat strains. As a consequence, treatment options studied for periods shorter than 6 months may have addressed mechanisms unrelated to the underlying pathogenesis. Pericyte dropout when assessed as pericyte “ghosts” succeeds also at 6 months of diabetes duration, but is identified earlier when quantitative retinal morphometry by image analysis is used [14]. Microaneurysms occasionally develop in the diabetic rat retina, but after extended disease duration (difficult to achieve in diabetic rats). No other lesions develop reproducibly. Diabetic mice of either type of diabetes develop similar lesions within comparable time frames as do rats, but the degree of cellular change is, to some extent, smaller, and gender effects on glycemia and retinopathy development may be stronger.
12.7 Mechanisms of Diabetic Microvascular Damage
The ability of a cell to cope with high ambient glucose is a crucial determinant to explain differential susceptibility of organs towards hyperglycemic damage. Vascular endothelial cells do not show changes in glucose transport rates when ambient glucose is high, which can result in elevated intracellular glucose [15]. The biochemical consequences of this phenomenon have become known as the “unifying hypothesis” about which excellent reviews have been published [16, 17]. For the purpose of this chapter, a brief summary is given here. High intracellular glucose leads to an increase in oxidative stress from different sources, including mitochrondrial overproduction of reactive oxygen species (ROS), enzyme mediated cytoplasmatic ROS production, and receptor mediated ROS generation. As the 50 or so different cell types of the retina are per se variably equipped with glucose transporters responding to the ambient glucose levels, with receptors responding to reactive intermediates and advanced glycation endproducts (AGEs), and enzymes producing ROS in the cytoplasm, it is likely that not one single mechanism is involved. Hyperglycemia activates four major pathogenetic pathways which may affect vascular cells in the diabetic retina: a. increased formation of AGEs and overexpression of its receptor, called RAGE (receptor for AGEs), b. activation of protein kinase C isoforms, c. activation of the polyol pathway, and d. activation of the hexosamine pathway. These mechanisms are the result of a single upstream event, i.e. mitochondrial overproduction of reactive oxygen species . Although some evidence exists that the activation of the polyol pathway is also relevant to microvascular changes in diabetes, glucose in diabetic vascular cells may not be a substrate for aldose reductase, as the Km of aldose reductase for glucose is 100 mM, while the intracellular concentration of glucose endothelial cells exposed to high glucose is in the nanomolar range [18]. In the retina of diabetic rodents, activation of the other pathways have been identified.
In general, AGEs form from glucose, glycolytic intermediates such as dicarbonyls, and from intermediates of free fatty acid oxidation. Extracellular AGEs form from glucose and have a slow formation characteristics, but are found in increased amounts in the extracellular matrix. Intracellular AGEs are rapidly formed from glycolytic intermediates such as methylglyoxal, and contributes to cell damage by three mechanisms: a. modification of protein function, b. alteration of cellular function via receptor recognition, and c. by changes in matrix interactions. AGEs can leak from producing cells to the plasma or accumulate on long-lived matrix molecules [19]. They can interact with the AGE receptor RAGE on various cells which activates the transcription factor NFkB. In the diabetic retina, RAGE has been identified on glial cells [20]. RAGE expression is regulated by high glucose in vitro and in vivo, involving methylglyoxal as a stimulator which increases NFkB and AP-1 binding to the RAGE promoter. AGEs like ROS can induce the overproduction of VEGF by retinal glia. AGEs can cooperate with the hexosamine pathway (see below) to modify transcriptional co-repressors changing transcriptional activation of growth factors [21]. Thus, AGEs can mimick hypoxia in the diabetic retina. On the contrary, AGEs may interfere with transactivation of HIF-1 alpha which mediates hypoxia-stimulated VEGF production. Covalent modification of the transcriptional coactivator p300 by hyperglycemia through methylglyoxal is responsible for the decreased association with HIF-1 alpha leading to reduced VEGF transcription [22]. The net balance of pro- versus antiangiogenic effects of AGEs in the diabetic retina may tip to the antiangiogenic site, since vasoregression dominates in the animal model in which these observations are made.
Several PKC isoforms are involved in cellular dysfunctions of the diabetic retina. Persistent PKC activation results from enhanced de-novo synthesis of diacylglycerol from hyperglycemia-induced triose phosphate which is available because mitochondrial ROS overproduction inhibitor the glycolytic enzyme GAPDH. PKC activation can also result from RAGE activation via AGEs. The role of PKCß activation affecting retinal blood flow and permeability has been established [23]. Specifically, VEGF activates PKC beta in the retina by membrane translocation and subsequent hyperpermeability, which can almost completely be blocked by ruboxistaurin. A novel association has been recently reported between pericyte loss and PKC δ [24]. PKC δ activation leads to increased expression of SHP-1, a protein tyrosine phosphatase affecting PDGF-ß receptor downstream signaling and pericyte apoptosis. PKC activation may also affect VEGF transcription which affects vacular permeability.
The fourth hyperglycemia-driven biochemical abnormality is the activation of the hexosamine pathway. Fructose-6-phosphate, diverted from glycolysis, is the substrate for fructose 6-phosphate amidotransferase (GFAT) and is converted into UDP-N-acetylglucosamine (GlucNac). Specific transferases utilize GlucNac for modification of serine and threonine residues. As mentioned, AGE modification of the transcriptional repressor mSin3A increases the recruitment of such a transferase resulting in the posttranslational modification of SP3, which results in a decreased binding to the glucose-sensitive GC box in the angiopoietin-2(Ang-2) promoter [21]. The resulting overexpression of Ang-2 precedes pericyte loss in the diabetic retina, and accelerates the formation of capillary dropout. Ang-2 has also been implicated in pericyte migration as an additional mechanism by which incipient pericyte loss in the diabetic retina can occur beyond apoptosis [25]. The hexosamin pathway was also reported to be relevant for TGF-ß1 and PAI-1 expression, however, of which the relevance in the diabetic retina has yet to be determined.
Given the inability to prevent complications by only targeting one of the above described abnormalities, a unifying mechanisms was introduced representing a common denominator of the biochemical abnormalities, and a mechanism by which hyperglycemic memory could be explained. This mechanism conceptually involves the overproduction of superoxide by the mitochondrial electron-transport chain. Intracellular excess glucose induces an abnormally high flux of electron donors into the mitochondrial electron transport chain. The resulting voltage gradient leaks superoxide because electron transfer is blocked at complex III of the electron chain. In endothelial cells exposed to excess glucose in vitro, superoxide production and subsequent ROS formation is increased. ROS overproduction is blunted by collapse of the gradient through UCP-1 or superoxide degradation by dismutase activity. “Rho zero” cells lacking mitochondria fail to produce ROS suggesting that mitochondria are an important source for ROS overproduction in glucose exposed cells. Mitochondrial ROS overproduction causes DNA damage which activates the repair/protection enzyme system called poly-ADP ribose polymerase (PARP). PARP which normaly resides in the nucleus in its inactive form, translocates to the cytoplasm upon ROS activation causing polymers of ADP-ribose to form. These polymers inhibit GAPDH activity which leads to an increase in the glycolytic intermediates which are the substrates for the biochemical pathways and thus lead to an overactivation. In the diabetic retina, ROS overproduction, PARP activation, increased availability of methylglyoxal, activation of the PKC and the hexosamine pathway have been detected [18, 26]. Several of these changes are readily reversible upon glucose normalisation but cannot explain findings from preclinical and clinical studies showing a lasting effect of hyperglycemia despite complete euglycemic restoration. For example, diabetic dogs developed similar degrees of retinopathy when treatment was changed from bad to good glycemic control. Clinically, patients on good glycemic control over 6.5 years in Diabetes Control and Complication Trial persisted to have much lower retinopathy progression during subsequent 14 years of follow-up, although their glycemia had become identical to former control patients whose glycemia had been much worse during the initial 6.5 years [27]. This phenomenon termed “hyperglycemic memory” is found in patients with type 2 diabetes (“hyperglycemic legacy”) and only glycemic control, but not blood pressure control produces such memory in the retina [28]. One cause for a memory effect is the covalent attachment of some AGEs to long-lived molecules such as extracellular matrix components. Another cause is the induction of sustained gene expression by glucose-mediated mitochondrial overproduction involving activating methylation of histones associated with critical gene promoters [29]. One example is the activation of the monomethyltransferase Set7 which was shown to be induced by transient high glucose levels. Sixteen hours of high glucose exposure resulted in 6 days of NF-kB transactivation. The Set7 activation led to a transcriptional activation of the p65 subunit of NF-kB (lasting for days) which induced proinflammatory cytokine production both in endothelial cells in vitro and in diabetic tissues in vivo. The permanent activation was inhibitable by superoxided dismutase and, importantly, by overexpression of glyoxalase-1 which is the critical enzyme to degrade methylglyoxal. Similarly, increased H4K3 methylation of the SOD2 gene causes downregulation of the enzyme activity, and failure to normalize the deficit upon euglycemic reentry suggests yet another potential role of epigenetic modifications, caused by ROS and AGEs in hyperglycemic memory. Other posttranslational modifications occurring in the diabetic setting comprise acylation, acetylation, prenylation and ubiquitination, as recently outlined [30].
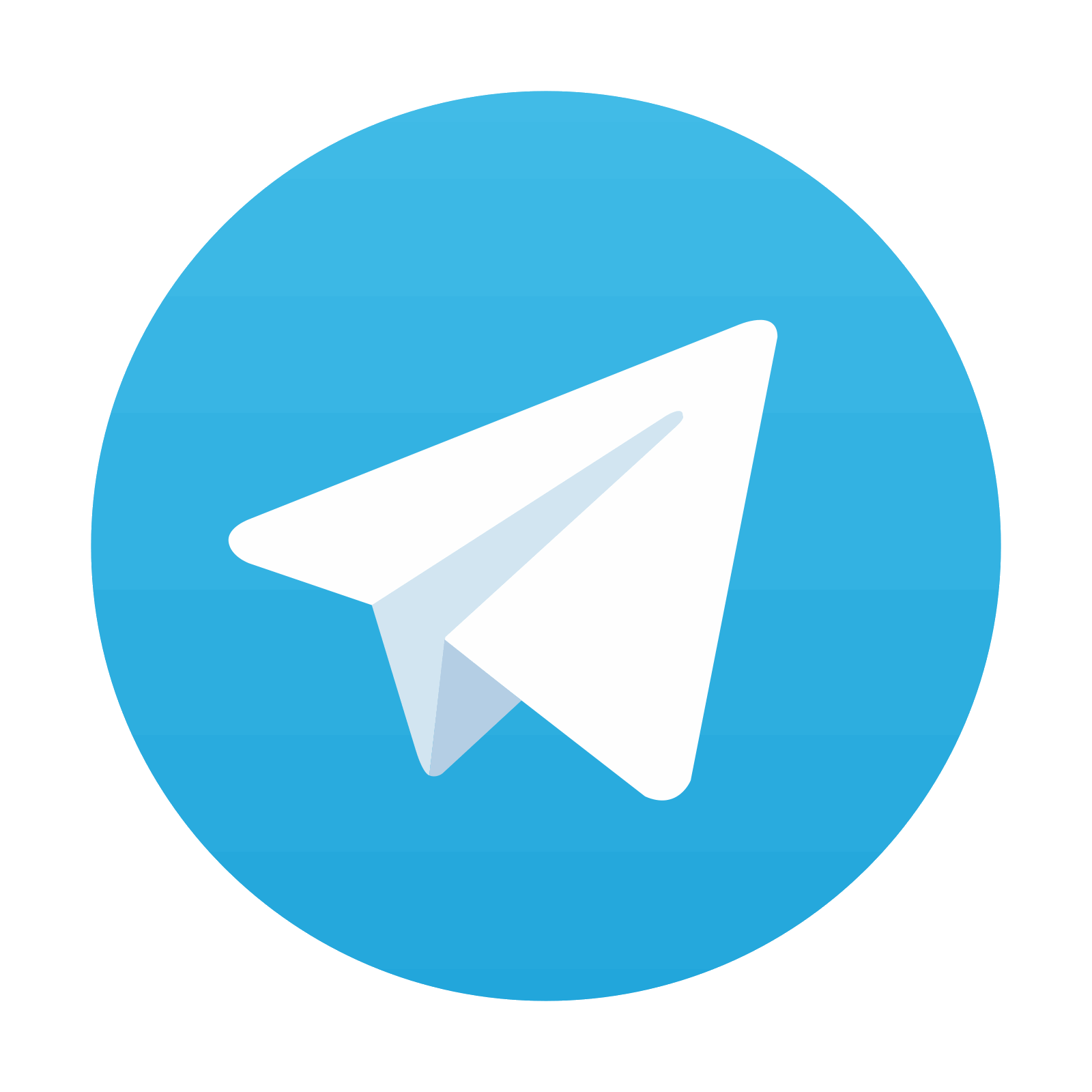
Stay updated, free articles. Join our Telegram channel
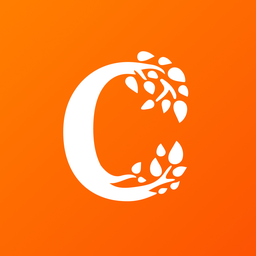
Full access? Get Clinical Tree
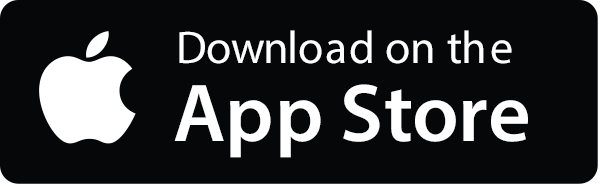
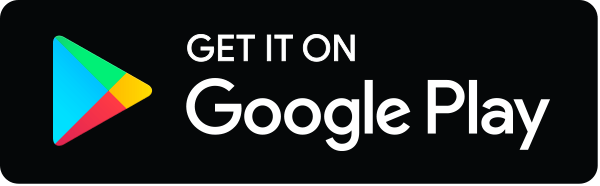