Fig. 10.1
a Distribution of tissue factor (TF) in the vessel wall. b Disturbance of the membrane asymmetry in the membrane of activated platelets enables Ca2+-dependent binding of coagulation factors to negatively charged phospholipids (phosphatidylserine) via γ-carboxyglutamic acid (Gla) residues situated in Gla domains
Coagulation can be started by two pathways (Fig. 10.2):

Fig. 10.2
Schematic view of the coagulation network subdivided in an initiation phase, a propagation phase, and a termination phase. Tissue factor, FVa, and FVIIIa act as cofactors to augment the zymogen activation of coagulation factors
1.
The intrinsic pathway, which is triggered by contact activation of coagulation factor XII.
2.
The extrinsic pathway, which is initiated by the transmembrane glycoprotein TF .
A substantial body of evidence implicates the contact pathway that has no essential role in hemostasis in the development of thrombosis (Fig. 10.2). The intrinsic pathway is initiated by factor XII in a reaction involving high molecular weight kininogen (HMWK) and plasma kallikrein (PK), collectively referred to as plasma contact system [10]. Contact with negatively charged surfaces induces a conformational change in FXII zymogen, resulting in small amounts of active FXII (FXIIa). FXIIa cleaves PK to generate active kallikrein, which in turn reciprocally activates additional FXII [11]. Physiologically relevant surfaces such as extracellular RNA [12] and platelet-derived polyphosphates [13] were shown to trigger FXII activation. FXIIa cleaves factor XI (FXI), leading to activation of FIX by FXIa in the intrinsic pathway (Fig. 10.2). Importantly, FXI activation is further augmented by thrombin via a feedback mechanism [14].
The coagulation initiator TF is constitutively expressed in highly vascularized organs such as the placenta, brain, heart, kidney, and lung [15]. TF is especially expressed in the adventitial and medial layers of the vessel wall by fibroblasts and vascular smooth muscle cells [16] (Fig. 10.1a). Endothelial cells under physiological conditions are probably devoid of TF [6], and TF was originally thought to be exclusively located in the extravascular compartment without any contact with the plasma coagulation factors. Perivascular TF thus forms a hemostatic envelope and is ready to activate coagulation when vascular integrity is perturbed [16]. In contrast, in thrombosis the initiation of blood coagulation can be triggered by TF that is present in human blood under physiological conditions [17]. This pool of TF has been termed circulating or blood-borne TF [18]. Microparticles that originate from monocytes were identified as major TF carriers in blood [19].
Class 2 of the cytokine receptor superfamily comprises the interferon-α receptor, interferon-γ receptor, interleukin (IL)-10 receptor, and the coagulation initiator TF [20]. The crystal structure of the extracellular region of the coagulation initiator TF revealed that it consists of two fibronectin type III-like domains with a binding site for FVII that lies at the interface region and involves residues from domain 1 and an extended loop of immunoglobulin-like domain 2 [21–23]. TF is the cofactor for the plasma serine protease factor VIIa. The procoagulant activity of factor VIIa dramatically increases after binding of its membrane receptor TF [24]. The TF/VIIa binary complex then activates the serine protease zymogens FIX and FX by limited proteolysis [25, 26]. Subsequently, FIXa binds its cofactor VIIIa and activates more FX in the so-called Xase complex (Fig. 10.2). Together with its cofactor FVa, FXa forms a complex on the surface of activated platelets, the so-called prothrombinase complex which activates prothrombin [27] (Fig. 10.2). Ca2+, together with negatively charged phospholipids (PS), mediates the anchoring of coagulation proteases on the surface of activated platelets, which is crucial for the formation of the functional coagulation complexes. The protease thrombin formed initially in coagulation is a pivotal enzyme [28] that activates FV, FVIII, and FXI, and thereby amplifies its own formation (Fig. 10.2). Cleavage of fibrinogen by thrombin leads to the formation of fibrin polymers (Fig. 10.2), and thrombin also activates FXIII, a transglutaminase that crosslinks fibrin and thus stabilizes the fibrin meshwork.
Thrombin is not only involved in the regulation of procoagulant mechanisms but also initiates fibrinolytic and anticoagulant systems that counteract fibrin formation when it binds to the vascular endothelium through specific receptors [29]. Thrombin bound to thrombomodulin on the endothelial cell surface has altered macromolecular substrate specificity and is no longer procoagulant, but now activates protein C [30]. Activation of protein C is augmented by the endothelial protein C receptor (EPCR) [31]. Activated protein C (APC) binds to protein S on the surface of activated cells and this complex then proteolytically inactivates factors Va and VIIIa, thus halting coagulation by disabling the formation of the prothrombinase (Va/Xa/prothormbin) and the intrinsic Xase complex (VIIIa/IXa/X) [32]. Furthermore, APC can exert additional anticoagulant action by inactivation of the tissue plasminogen activator inhibitor, thus enhancing fibrinolysis [33]. However, the protein C pathway not only regulates coagulation but the protein C /EPCR complex also activates protease-actiated receptor (PAR)-1 [34], and thereby inhibits inflammatory signaling and induces cell survival of endothelial cells [35–36].
10.2 Activation of Protease-Activated Receptors (PARs) by Coagulation Proteases
Thrombin , the central trypsin-like serine protease that converts fibrinogen to fibrin also promotes activation of PAR 1 and PAR4 on human platelets, and thus connects coagulation and platelet activation [37]. The first thrombin receptor (PAR1) was cloned in 1991 [38] and three other members of this receptor family have been identified [39–41] (Fig. 10.3). Members of the PAR family of heptahelical G-protein-coupled receptors (GPCRs) are expressed on various cell types, including endothelial cells, smooth muscle cells, fibroblasts, epithelial cells, mast cells, neutrophils, monocytes, and macrophages [42–48]. These receptors not only mediate the cellular actions of the central coagulation protease thrombin on platelets but they also fulfill important nonhemostatic functions in development, play a role in tumor biology, regulation of inflammatory responses, and mediate remodeling and tissue repair processes. In contrast to human platelets, mouse platelet thrombin signaling is mediated via PAR3 and PAR4 [49], and therefore phenotypes of PAR1-deficient animals reflect only on PAR1 signaling in extravascular and vascular cells other than platelets. In addition to their function in thrombosis and hemostasis , the coagulation proteases and their cellular receptors are involved in a myriad of vascular signaling processes that ensure the maintenance of vascular development , endothelial function, and vascular tone [50].

Fig. 10.3
Cleavage sites of serine proteases at the N-terminus of protease-activated receptor (PAR)-1
The initiation complex of coagulation that is formed by the membrane receptor TF and its ligands, the coagulation proteases Factor VIIa and Factor Xa, elicits the proteolytic activation of PAR2, whereas Factor Xa also activates PAR1 [51]. The seven-transmembrane GPCRs PAR1 and PAR2 serve partially redundant functions as vascular detectors of coagulation activation [52]. In the vasculature, expression of the thrombin receptors PAR1 and PAR2 was detected on human endothelial cells, smooth muscle cells, and macrophages [43, 53–56]. PAR1 is required for developmental angiogenesis [57–59]. In contrast, PAR2 signaling regulates postnatal and pathological angiogenesis [60, 61]. Furthermore, PAR1 and PAR2 are involved in microvascular remodeling [62, 63]. Dysregulation of PAR-mediated coagulation factor signaling under pathologic conditions results in increased angiogenesis (e.g. in malignancy of solid tumors) [64] or an augmented innate immune response (e.g. during the pathogenesis of sepsis or viral infections) [65, 66]. Furthermore, activation of PAR1 and PAR2 signaling lowers vascular tone via activation of the nitric oxide (NO)-synthase pathway [67]. The regulation of vascular tone [43] and permeability [68, 69] is predominantly caused by PAR signaling in endothelial cells.
The PARs are activated via limited proteolysis by serine proteases close to the N-terminus of the receptor [48] (Fig. 10.3). The newly formed N-terminus functions as a tethered peptide agonist that binds intramolecularly to the seven-transmembrane helix bundle of the receptor, and thus affects G-protein activation. Transactivation of another PAR has also been documented for PAR1 or PAR3 which have tethered ligands capable of activating PAR2 in heterodimeric complexes [70–72].
The G-protein-coupled PAR downstream signaling can be exemplified with PAR1, which couples to members of the G12/13, Gq, and Gi families [73–75]. The α-subunits of G12 and G13 bind Rho guanine-nucleotide exchange factors that activate small G-proteins and induce Rho-dependent cytoskeletal responses involved in the platelet shape change [76], the regulation of permeability [77], and migration of endothelial cells [78]. Gαq activates phospholipase Cβ and triggers the hydrolysis of phosphoinositides, resulting in calcium mobilization and activation of protein kinase C. This activates Ca2+-regulated kinases, phosphatases, guanine-nucleotide exchange factors, and mitogen-activated protein kinases (MAPKs), resulting in cellular responses that range from granule secretion to integrin activation, aggregation and transcriptional responses in endothelial and mesenchymal cells [79]. Gαi inhibits adenylate cyclase and thus promotes platelet responses. Gβγ subunits can activate phosphoinositide 3-kinase (PI3K) and other lipid-modifying enzymes, protein kinases, and ion channels [80, 81]. Thus, PAR1 activation results in pleiotropic effects and the diverse actions which thrombin exerts on different cell types [49].
Several serine proteases can activate PAR1 at the same cleavage site (i.e. the Arg41–Ser42 bond) (Table 10.1). For instance, the primary protease that activates PAR1 is thrombin, but the receptor is also activated by FXa and the anticoagulant serine protease APC [51]. However, APC has effects opposite to thrombin in barrier protection, regulation of endothelial inflammation, and apoptosis [82, 83]. These distinct signaling properties have recently been shown to be caused by biased agonism, since APC, but not thrombin , can also cleave PAR1 at Arg46, generating an alternative tethered ligand sequence [82, 84]. Thrombin cleavage of PAR1 results in proinflammatory effects, whereas the N-terminus of PAR1 that is generated by APC cleavage at Arg46 acts as a biased agonist for cytoprotective effects [84]. Matrix metalloproteinases (MMPs) also activate PAR1 at a distinct cleavage site [85, 86], but it is unclear whether this produces a biased agonist response, as shown for APC cleavage.
Table 10.1
The activating proteases of protease-activated receptors (PARs)
Activating proteases |
PAR1 |
PAR2 |
PAR3 |
PAR4 |
---|---|---|---|---|
Coagulation and fibrinolysis system |
Thrombin
Plasmin
FVIIa, FXa
Activated protein C |
FVIIa, FXa
Activated protein C |
Thrombin |
Thrombin plasmin |
Others |
Trypsin
Tryptase
Cathepsin G
Granzyme A
Matrix metalloproteinase-1
Gingipain-R |
Trypsin I, IV
Mast cell
Tryptase
Matriptase
Epitheliasin
Proteinase 3
Acrosin
Kallikrein 5, 6, 14 |
Trypsin
Cathepsin G
Gingipain-R |
Several proteases activate a number of PARs; for example, APC can activate both PAR1, PAR2 [87], and PAR3 [71]. PAR cleavage does not necessarily lead to receptor activation if cleavage occurs at a site that amputates the tethered ligand sequence (e.g. Cathepsin G cleavage of PAR1). The proteases that either activate or inactivate PARs are listed in Table 10.1 and Table 10.2, respectively [50, 88].
Table 10.2
The inactivating proteases of protease-activated receptors (PARs)
Inactivating proteases |
PAR1 |
PAR2 |
PAR3 |
PAR4 |
---|---|---|---|---|
Coagulation and fibrinolysis system |
Plasmin |
Plasmin |
||
Others |
Trypsin
Cathepsin G
Elastase
Chymotrypsin |
Plasmin
Cathepsin G
Proteinase 3
Elastase |
Cathepsin G
Elastase |
10.3 Coagulation Factor and PAR1 Deficiency in Genetic Mouse Models Results in Arrested Vascular Development
The central role of the initiation of coagulation by TF and of thrombin generation for embryonic development of a functional vasculature has been demonstrated with mice that are deficient in coagulation factors and with mouse models that lack PARs. TF plays an indispensable role in establishing and maintaining vascular integrity in the embryo at days 9.5–10.5 when embryonic and extraembryonic vasculatures initially form and fuse (Fig. 10.4a). Targeted disruption of the murine TF gene results in defective yolk sac vessels and embryos, with severe growth and development retardation at day E10.5 [89, 90]. Approximately 85 % of the TF−/− embryos die before E11.5, and embryos that manage to escape this developmental bottleneck do not survive gestation [90]. Mouse embryogenesis critically depends on the TF extracellular domain but is independent of its cytoplasmic domain [91]. Day E9.5 TF−/− embryos showed extremely pale yolk sacs, highly enlarged pericardial sacs, and poorly developed forebrain structures. Embryonic red blood cells are not retained in the yolk sac vessels in TF−/− embryos, suggesting that TF is required to maintain vascular integrity [90, 92]. At E10.5, TF−/− embryos were significantly smaller than their TF+/− and TF+/+ littermates [92]. Interestingly, plasma clotting time and bleeding time of TF+/− mice was similar to TF+/+ mice, suggesting that half-normal amounts of TF are sufficient for both hemostatic function and vascular development [91]. The TF cytoplasmic domain contains a conserved protein kinase C phosphorylation site [93] that is phosphorylated by p38 (X-S*/T*-P-X) [94]. However, deficiency of the TF cytoplasmic domain does not result in abnormal embryonic development [95]. This further supports the notion that the cofactor function of the extracellular domain is critical for vascular development .

Fig. 10.4
Similar vascular phenotypes of tissue factor (TF), FX, thrombin, and protease-activated receptor (PAR)-1-deficient mouse embryos. Vascular development in mice with defective thrombin signaling is perturbed between E9.5 and E12.5, leading to vascular leakage, growth retardation, and embryonic lethality. a Day E10.5 TF+/+ (left) and TF−/− (right) embryos dissected from their yolk sacs. An obvious developmental arrest was noted with pale appearance, massively enlarged pericardial sac (white arrow heads) and distended heart (black arrow head) of the mutant embryo ([92]). b Embryos deficient in FX show normal gross morphology compared with wild-type controls at E11.5 and E12.5 but indicate the occurrence of bleeding ([96]). c E12.5 embryos deficient in prothrombin (FII) showed pale appearance and developmental arrest. d PARcharged phosphatidylserine supporting binding1−/− (F2r−/−) embryos at E10.5 (d3Only PAR1) show developmental delay and dilated, blood-filled pericardial cavity compared with PAR1+/− (F2r+/−) littermate embryos (d1). β-Galactosidase-stained yolk sacs of PAR1+/− embryos (d2) and PAR1−/− embryos (d4) carrying a TIE2p/e-LacZ transgene that allows visualization of the vascular endothelium. Scale bars, 1 mm
Similar to TF−/− mice, mice that are deficient in coagulation factor X suffer partial embryonic lethality between E11.5 and E12.5 and fatal neonatal bleeding [96] (Fig. 10.4b). At day E12.5, the percentage of non-resorbed FX−/− embryos was only 17 %. The majority of factor X−/− neonates die before postnatal day 5, and show intraabdominal, subcutaneous, or massive intracranial bleeding. In contrast to TF−/− mice, embryos deficient in FVII develop normally but succumb to early intra-abdominal or intracranial bleeding later in life [97]. However, survival of FVII-deficient embryos has been shown to be due to materno-fetal transfer of FVII [98].
Fifty percent of factor V-deficient embryos lacking the cofactor that is required to form the prothrombinase complex die at mid-gestation with bleeding and vascular abnormalities in the yolk sac [99]. Those FV−/− embryos that survive the developmental arrest suffer fatal postnatal bleeding. Prothrombin-deficient mice (FII−/−) also show partial embryonic lethality, with more than one-half of the FII−/− embryos dying between E9.5 and E11.5 [100] (Fig. 10.4c). Again, bleeding into the yolk sac cavity was observed. One-quarter of the FII−/− mice survived to term but died within a few days after birth due to hemorrhage. Since fibrinogen-deficient embryos [101], as well as NF-E2-deficient embryos with very low platelet counts [102], do not show signs of abnormal development, it is unlikely that defective clot formation is responsible for the impaired vascular development observed in TF, FX, FV, and prothrombin-deficient mice. The similarity of embryonic phenotypes of FV, FX, and prothrombin-deficient mice suggests a pivotal role of thrombin generation and formation of the prothrombinase complex (FVa/FXa/prothrombin) in embryonic vascular development .
Only PAR1−/− mice (F2r−/− thrombin-receptor-deficient mice) show vascular defects resulting in embryonic death, whereas no abnormalities in vascular development were observed in PAR2−/−, PAR3−/−, or PAR4−/− mice [50]. Until gestational day E8, PAR1−/− mice develop normally but their growth is retarded at E9 compared with PAR1+/− control mice [103]. At E9.5, gross bleeding (22 %) and microscopic bleeding (66 %) becomes apparent, and half of the PAR1−/− embryos die due to vascular defects (Fig. 10.4d) and half survive with no apparent defects in the vasculature [56]. Similar to the coagulation factor knockouts, day E9.5 PAR1−/− embryos with development abnormalities have yolk sacs that lack blood-filled vessels (Fig. 10.4d). A dilated pericardial sac is observed in embryos with bleeding, a sign of cardiovascular failure. Pericardial bleeding becomes prominent at E10.5, and 52 % of PAR1−/− embryos die by day E12.5 [57]. Endothelium-specific expression of PAR1 under control of the TIE2 promoter prevented embryonic death of PAR1-deficient mice. This suggests that PAR1 signaling in endothelial cells is crucial for normal vascular development [57].
The similar vascular phenotypes of TF, FX, FV, prothrombin, and PAR1-deficient mice indicate that thrombin-mediated signaling via endothelial PAR1 is indispensable for embryonic vascular development at mid-gestation [57]. The central role of thrombin-mediated PAR1 signaling in embryonic blood vessel development is further supported by the finding that combined deficiency of PAR4, required for platelet activation, and fibrinogen recapitulates the hemostatic defect of prothrombin-deficient mice but not the early embryonic lethality that also characterizes prothrombin-deficient mice [104]. In addition to vascular development, partially redundant signaling of PAR1 and PAR2 is required for neural tube closure in the mouse embryo which is mediated by a local network of membrane-tethered proteases [105].
10.4 Regulatory Mechanisms of Coagulation Factor Signaling
Binding of FVII to TF, the allosteric catalytic activation of FVIIa, and negatively charged phosphatidylserine supporting binding of γ-carboxyglutamyl residues in the Gla domains of substrates provide effective mechanisms for localizing coagulation initiation to membrane surfaces. The procoagulant function of TF is tightly regulated by the TF pathway inhibitor (TFPI) [106], the physiologic inhibitor of the initiator complex (TF/VIIa/Xa). The Kunitz domain 2 of TFPI forms a complex with FXa, and Kunitz domain 1 with FVIIa, and TFPI thus inhibits coagulation initiation as well as TF-induced signaling via PARs [107]. However, the Kunitz-type inhibitor TFPI is prone to degradation by leukocyte serine proteases [108]. It can be degraded by neutrophil elastase and cathepsin G in neutrophil extracellular traps (NETs) of platelet-neutrophil conjugates [4] .
TF function is also regulated by post-translational modifications of the receptor [109]. TF has two disulfide bridges at positions Cys49–Cys57 and Cys186–Cys209. The Cys186–Cys209 disulfide is required for function [110]. It lies at the end of the fibronectin type III-like domain 2 and links adjacent strands of an antiparallel β sheet [21]. This disulfide bond is exposed to solvent and is prone to reduction [21]. TF with a reduced Cys186–Cys209 disulfide has very low procoagulant activity but can be converted to the functional receptor by formation of the C186–C209 disulfide bond by oxidoreductase-mediated dithiol-disulfide exchange reactions [111–113]. The redox state of the TF Cys186–Cys209 disulfide determines the signaling specificity of the binary complex (TF/VIIa) [111]. Whereas signaling of the binary complex (TF/VIIa) can occur with a broken TF Cys186-Cys209 disulfide bond, ternary complex signaling (TF/VIIa/Xa) requires this disulfide [111] .
TF interacts with α3β1 and α6β1 integrins [114], and integrin ligation of TF has been shown to support TF/VIIa proteolytic signaling through PAR2 [115]. In contrast to full-length TF, the alternatively spliced variant of TF regulates angiogenesis independent of PAR2 and FVIIa by direct integrin ligation of αvβ3 or α6β1 [116]. Integrin function is also regulated by the TF cytoplasmic domain. PAR2 activation by a PAR2 agonist and TF signaling complexes leads to phosphorylation of the cytoplasmic domain of human TF at position Ser 253 and Ser 258 [116]. The phosphorylation of Ser 253 requires PKCα [117], and p38 phosphorylates Ser 258 [94], in a process that is regulated by thioester modification of TF at the intracellular Cys 245 residue [118]. TF cytoplasmic domain signaling regulates integrin function in cell migration. It can exert negative effects as well as TF/FVIIa and phosphorylation-mediated positive effects [114] in postnatal angiogenesis [62, 63] .
10.5 PAR Signaling Regulates Expression of Angiogenic Factors and Vascular Function
The (patho)physiologic responses of coagulation factor-activated PAR signaling in the vasculature are mediated via changes in the expression profile of a myriad of autocrine and paracrine factors in a cell-type-specific manner.
10.5.1 Cellular Effects of PAR1 Signaling on Angiogenesis
Endothelial Cells
Thrombin induces PAR1-dependent angiogenesis in various angiogenesis models (e.g. chick chorioallantoic membrane assay, matrigel plug assay) [119, 120]. In endothelial cells [121, 122], thrombin exerts mitogenic and antiapoptotic effects via PAR1-induced release of heparin-binding epidermal growth factor (HB-EGF) [123]. Thrombin treatment is accompanied by the induction of vascular endothelial growth factor (VEGF) and angiopoietin-2 (Ang2) transcripts [119], and by potentiation of VEGF activity on endothelial cells by upregulation of its receptor VEGFR-2 [124]. Thrombin stimulation upregulates growth-regulated oncogene-α in endothelial cells, and this chemokine can induce the expression of VEGF, Ang2, and VEGFR-2 [125].
In human late endothelial progenitors, PAR1 activation affects angiogenesis by upregulating the SDF-1/CXCR4 system [126]. In addition to its effects on cells of the vascular wall, thrombin also regulates the expression of proangiogenic cytokines via activation of PAR1 in mononuclear cells [127]. Moreover, PAR1-independent effects of thrombin on cytoprotection are mediated by α5β3 and α5β1 integrins [123]. Thrombin stimulation upregulates αvβ3 expression in human umbilical vein endothelial cells (HUVECs), and thus contributes to endothelial cell adhesion, migration, and survival [128].
Vascular Smooth Muscle Cells
PAR1 activation by thrombin increases the release of VEGF in human vascular smooth muscle cells. This effect is dependent on the intracellular Ca2+ concentration and on extracellular signal-regulated kinase (ERK1/2) signaling [129]. Hypoxia-inducible factor (HIF)-1α, a regulator of VEGF induction [130], can be upregulated under nonhypoxic conditions by a thrombin-dependent mechanism involving reactive oxygen species production in endothelial cells and smooth muscle cells [131, 132].
10.5.2 Cellular Effects of PAR2 Signaling on Angiogenesis
Endothelial Cells
Angiogenesis is supported by PAR2 signaling in several angiogenesis models (hindlimb ischemia, mouse retina model, matrigel assay) [61, 133, 134]. Incubation of HUVECs with a PAR2 agonist peptide elicits a dose- and time-dependent mitogenic response [135]. Small interfering RNA (siRNA) silencing of TF in human microvascular endothelial cells inhibits the formation of stable, tube-like structures, whereas overexpression of TF, or stimulation with a PAR2 agonist, rescue the loss of TF in a matrigel assay [134]. In endothelial cells, TF can regulate PAR -mediated microvessel formation by induction of chemokine ligand 2 (CCL2 or monocyte chemotactic protein-1 [MCP-1]) [136]. Of note, CCL2 is typically induced by PAR1 signaling [34]. In this in vitro assay, CCL2 mediates the angiogenic effect of TF by recruiting smooth muscle cells toward endothelial cells, and facilitates the maturation of newly formed microvessels. In the retinal vasculature, the proangiogenic properties of PAR2 depend on TNF-α and subsequent induction of the receptor tyrosine kinase TIE2 via the MEK/ERK pathway [137]. Furthermore, in FVIIa-stimulated HUVECs overexpressing TF and PAR2, the transcription factor cyclic AMP responsive binding protein (CREB) is phosphorylated and is thus activated [138].
10.5.3 Cellular Effects of PAR Signaling on Vascular Function
PAR1 Signaling
In addition to angiogenesis and vascular remodeling , PAR signaling influences vascular function through effects on both endothelium and vascular smooth muscle cells. Treatment of confluent HUVEC monolayers with thrombin leads to a change in the shape of the endothelial cells, resulting in gaps in the monolayer and exposure of the subendothelial matrix [139]. TFLLRNPNDK, a PAR1-selective activating peptide, caused hypotension and heart rate decreases in wild-type mice. These effects were absent in PAR1−/− mice [59]. The stimulation of PAR1 induces endothelium-dependent relaxation in human coronary artery rings, but the endothelium-dependent relaxation was attenuated with the severity of atherosclerotic lesions [140]. PAR1 activation changes endothelial expression of endothelin-converting enzyme (ECE) [141]. Furthermore, thrombin may induce NO production in endothelial cells [142]; however, long-term treatment of endothelial cells with thrombin downregulates endothelial NO synthase (eNOS) expression [141, 143].
PAR2 Signaling
PAR2 activation by the selective activating peptides SLIGKV-NH2 or SLIGRL cause arterial and venous dilation, leading to hypotension in humans and mice [59, 67, 144]. The SLIGRL-induced response was absent in PAR2-deficient mice, and these effects are reduced by inhibition of NO or prostaglandin synthesis.
10.6 Roles of PARs in Physiologic and Pathologic Processes
10.6.1 PAR Signaling in Wound Healing and Remodeling
The vascular phenotypes of PAR1-deficient mice clearly demonstrate an important role of coagulation factor-mediated PAR1 signaling in developmental angiogenesis [145]. In addition to developmental angiogenesis, PAR1-dependent signaling is also relevant in postnatal vascular remodeling of the small intestinal mucosa where epithelial coagulation factor signaling and PAR1 expression is triggered by the gut microbiota and upregulates expression of angiopoietin-1 (Ang1) [63]. Furthermore, activation of PAR signaling improves wound healing in the skin [146–149], and a proangiogenic function of APC that promotes cutaneous wound healing has been demonstrated [150]. Upon myocardial infarction, stimulation of PAR2 signaling alters the endothelial cell phenotype and induces expression of angiogenic chemokines [151]. Interestingly, PAR2-deficient mice were protected from postinfarction remodeling and showed less impairment of heart function [152]. PARs also contribute to fibrotic processes in a number of organs [153–155]. In cardiac fibroblasts, PAR1 signaling leads to transactivation of the EGF receptor (EGFR) pathway, which may contribute to cardiac remodeling [156].
10.6.2 PAR Signaling in Malignancy
A prothrombotic state and cancer-associated thrombosis is frequently observed in cancer patients [157], and TF procoagulant activity is crucial for metastatic tumor dissemination [158, 159]. Coagulation factor signaling via PARs promotes neovascularization and tumor cell migration, and thus contributes to growth and metastasis of many solid tumors [160–162] .
Expression and activation of PAR1 has been associated with tumor progression in prostate cancer, breast cancer, lung cancer, gastric cancer, colon cancer, melanoma, and ovarian cancer [163–170]. Breast carcinoma cell invasion is promoted by PAR1 activation, leading to persistent transactivation of EGFR and ErbB2/HER2 [171]. Inhibition of PAR1 signaling results in inhibition of Akt survival pathways in breast cancer cells, and thus suppresses tumor survival and metastasis [172]. Microarray studies have been performed to assess the transcriptional program induced via PAR1- and PAR2-dependent coagulation factor signaling [173, 174]. In this context, PAR1 mediates the induction of IL-8 and VEGF in prostate cancer cells [175]. PAR2 activation regulates VEGF and IL-8 expression in the breast cancer cell line MDA-MB-231 cells via MAPK pathways [176, 177]. Both the TF/VIIa/Xa complex and PAR2 are essential for FVIIa- and FXa-induced signaling, migration, and invasion of breast cancer cells [178, 179]. Formation of the TF/VIIa/Xa complex induces activation of the mammalian target of rapamycin (mTOR) pathway that regulates migration of human breast cancer cells [180]. In breast cancer development, cooperation of TF cytoplasmic domain and PAR2 signaling occurs [181], and TF phosphorylation correlates with PAR2 expression and is associated with relapse, indicating prognostic significance [182] .
10.6.3 PAR Signaling in Innate Immunity
Recent studies have demonstrated that PAR signaling plays a role in the regulation of the innate immune response. In a mouse model of LPS-induced sterile infection, PAR1-mediated coagulation factor signaling amplifies inflammation and lethality through sphingosine-1-phosphate downstream signaling in dendritic cells [65]. It has recently been shown that PAR1 contributes to the innate immune response during viral infection [183]. Furthermore, it has been demonstrated that TF-dependent PAR2 signaling on dendritic cells suppresses antigen-specific CD4+ T-cell priming [184]. In gingival epithelial cells, proteases of Porphyromonas gingivalis induce human β-defensin-2 expression via epithelial PAR2 activation [185]. MyD88-dependent, PAR2 agonist peptide-induced nuclear factor-kappa B (NFĸB) signaling is augmented by agonist peptide-dependent physical interaction of toll-like receptor 4 (TLR4) with PAR2 [186]. Interestingly, in high-fat diet-induced obesity the genetic ablation of TF-dependent PAR2 signaling reduced adipose tissue macrophage inflammation, and inhibition of macrophage TF signaling ameliorated insulin resistance [187]. This indicates a role for coagulation factor signaling in metabolic syndrome development .
10.7 Perspective
While the essential role of TF as a coagulation initiator is firmly established, the coagulation cascade plays important additional roles in embryonic and postnatal angiogenesis through the activation of PAR. More recent data expand this view and document much broader roles of coagulation protease signaling in physiological and pathological processes in the context of vascular diseases , wound healing, tissue remodeling, and cancer. The role of coagulation factor-mediated PAR signaling in the regulation of innate and adaptive immunity under various conditions is yet to be defined. In future, these areas of investigation will be aided by an increasing number of excellent molecular tools and genetic animal models.
References
1.
Furie B, Furie BC. Mechanisms of thrombus formation. N Engl J Med. 2008;359:938–49.PubMed
2.
Broze GJ Jr. Tissue factor pathway inhibitor. Thromb Haemost. 1995;74:90–3.PubMed
3.
Esmon CT, Esmon NL. The link between vascular features and thrombosis. Annu Rev Physiol. 2011;73:503–14.PubMed
4.
Massberg S, Grahl L, von Bruehl ML, Manukyan D, et al. Reciprocal coupling of coagulation and innate immunity via neutrophil serine proteases. Nat Med. 2010;16:887–96.PubMed
5.
Opal SM, Esmon C. Bench-to-bedside review: functional relationships between coagulation and the innate immune response and their respective roles in the pathogenesis of sepsis. Crit Care. 2003;7:22–8.
6.
Østerud B, Bjørklid E. Sources of tissue factor. Semin Thromb Hemost. 2006;32:11–23.PubMed
7.
Ruggeri ZM. The role of von Willebrand factor in thrombus formation. Thromb Res. 2007;120:S5–9.PubMedCentralPubMed
8.
Stegner D, Nieswandt B. Platelet receptor signaling in thrombus formation. J Mol Med. 2011;89:109–21.PubMed
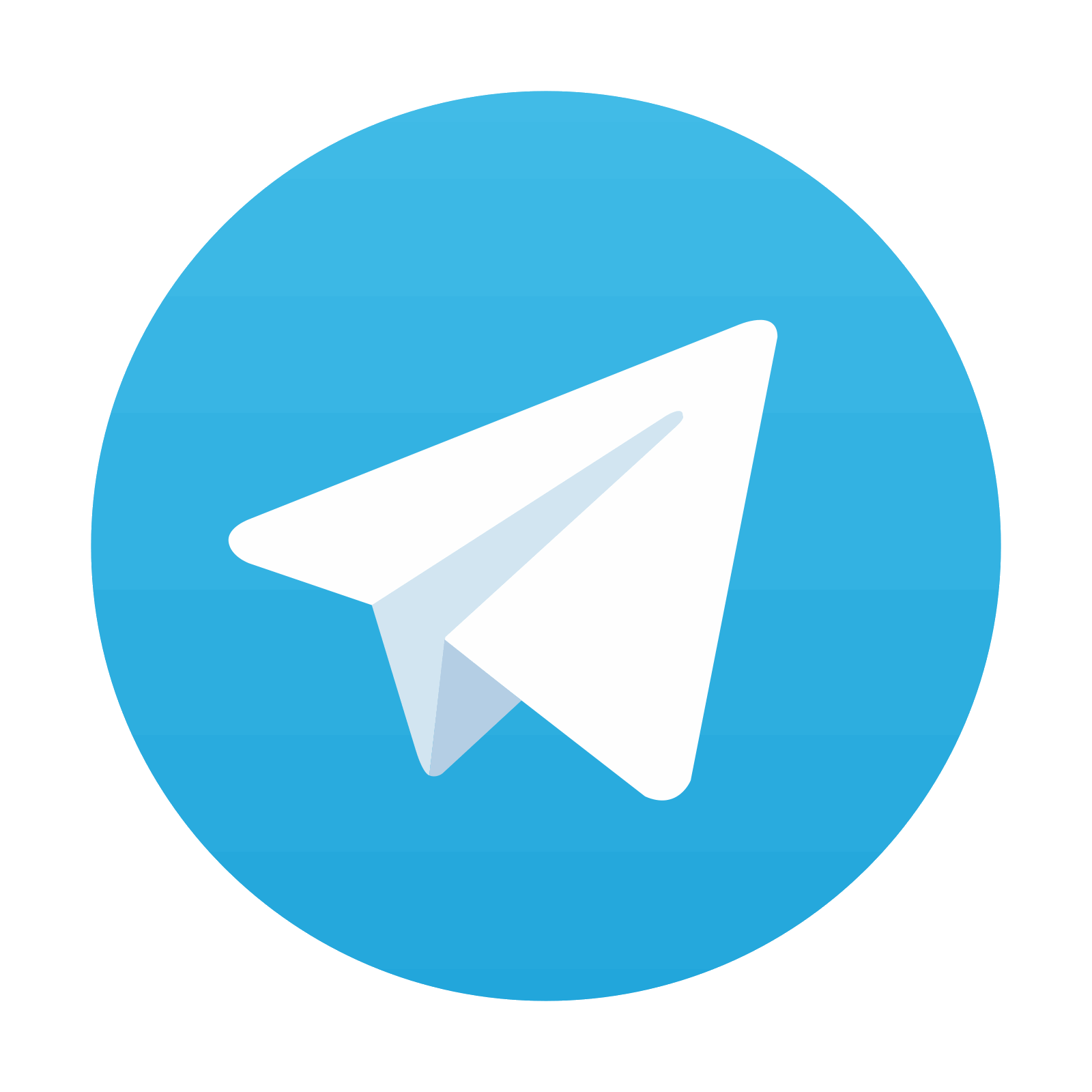
Stay updated, free articles. Join our Telegram channel
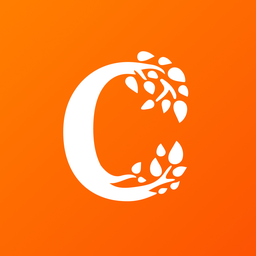
Full access? Get Clinical Tree
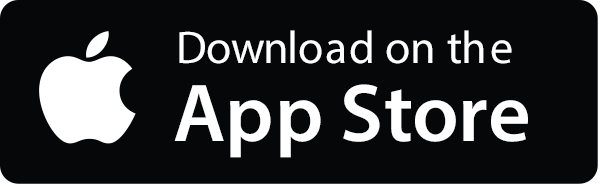
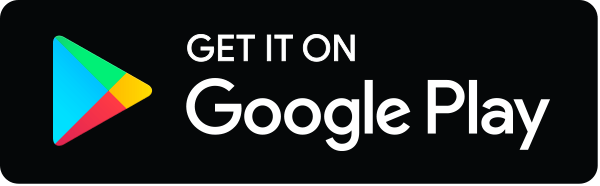