Fig. 15.1
Selection of possible hypoxia-dependent mechanisms contributing to pulmonary vascular remodeling in chronic obstructive pulmonary disease-pulmonary hypertension (COPD-PH). Cigarette smoke-induced airway obstruction and emphysema can result in hypoxia. Hypoxia, amongst others, activates hypoxia-inducible factor (HIF)-1α, which can trigger pathways associated with inflammation, the recruitment of bone-marrow-derived cells, and alterations in proliferation/apoptosis balance of vascular endothelial and smooth muscle cells (SMCs). Increased proliferation of SMCs causes narrowing of the vessels, resulting in pulmonary hypertension. All important acronyms of the molecules are explained in the text

Fig. 15.2
Hypoxia-induced ion channel-mediated increase in proliferation, contraction, and decrease of apoptosis of smooth muscle cells (SMCs) contributing to pulmonary vascular remodeling. K v and TASK-1 channels are downregulated and are less active after chronic hypoxia, leading to accumulation of K + within the cell (mediating apoptosis resistance) and membrane potential depolarization of the SMCs. This depolarization causes opening of voltage-operated Ca 2+ channels (VOCC), especially L-type channels, which mediate Ca 2+ entry. Hypoxia-dependent reactive oxygen species (ROS) regulation derived from nicotinamide adenine dinucleotide phosphate (NADPH) oxidases and/or mitochondria is suggested to inhibit the K + channels, although it is unclear whether an increase or decrease of ROS occurs in hypoxia. Transient receptor potential channel (TRPC)-mediated Ca 2+ or Na + influx (speculatively by gating K+ channels) was also shown to be essential for the intracellular Ca 2+ increase in at least acute hypoxia. Ca 2+ release from mitochondria and sarcoplasmic reticulum (SR) was shown to additionally increase Ca 2+ within the cell. Thus, mediated contraction and proliferation of the SMCs can contribute to vascular remodeling. Colored arrows depict either activation (green) or inhibition (red)
There are many cytokines mediating inflammation but, in the context of PH, special attention was given to HIF-1α-induced interleukin (IL)-6 because its messenger RNA (mRNA), as well as protein levels, were upregulated by chronic hypoxia in mice. Although IL-6-deficient mice showed less inflammation under hypoxic conditions, chronic hypoxic pulmonary hypertension (CHPH) was, however, only marginally reduced [71]. In contrast, IL-6 overexpression, specifically in the lungs, showed enhanced muscularization of small pulmonary vessels and PH under normoxic conditions, indicating that IL-6 may contribute to vascular remodeling in lungs. Of interest, overexpression of IL-6 under hypoxia resulted in severe PH and vascular remodeling up to obliteration [72]. Taken together, IL-6 is upregulated after chronic hypoxia in mouse lungs, but does not seem to be essential for CHPH development. Moreover, the combination of excessive IL-6 and chronic hypoxia seems to change the mode of vascular remodeling towards angioproliferation [49]. Based on recent results, there are currently two theories put forth to explain this observation. The first is that IL-6 is able to exert its effects through modulation of ‘bone morphogenetic protein receptor type 2’ (BMPR2) through a signal transducer and activator of transcription 3 (STAT3)-microRNA cluster 17/92 pathway [73]. BMPR2 is a member of the transforming growth factor (TGF)-β superfamily of growth factor receptors. It is expressed ubiquitously and is a member of many different signaling pathways, including pERK, JNK, Akt/PI3K [74, 75], pSmad1/5 [76], and p-p38MAPK [77]. Mutations in the BMPR2 gene are shown to be linked to PH development [78], and, moreover, BMPR2 expression is reduced in the pulmonary vasculature in primary PH patients [79]. The other prevailing theory is based on the observation that IL-6 can influence the balance between apoptosis and proliferation in pulmonary arterial SMCs (PASMC) and ECs (PAECs), leading to vascular remodeling. Overexpression of IL-6 induces the angioproliferative growth factor VEGF and intracellular extracellular signal-regulated kinase (ERK), resulting in increased proliferation. Simultaneously, IL-6 downregulates the growth inhibitor TGFβ and proapoptotic mitogen-activated protein kinases (MAPKs) [JNK1, p38MAPK], and upregulates the inhibitors of apoptosis Bcl-2 and survivin, leading to decreased apoptosis [72]. In conclusion, inflammatory cytokines, especially IL-6, can trigger vascular remodeling by influencing signaling pathways which lead to more proliferation and less apoptosis of PASMCs and PAECs (Fig. 15.1).
Furthermore, HIF-1α can induce PASMC depolarization by reduction of K+ channel expression and activity that is associated with intracellular K+ accumulation, and increase intracellular Ca2+ concentration and pH by upregulating transient receptor potential channels (TRPC) and Na+/H+ exchanger [80–83], (Fig. 15.2). It has been shown that the currents of voltage-gated K+ channels (Kv) are decreased under chronic hypoxic conditions [84–86], most likely mediated by reactive oxygen species (ROS) derived from mitochondria [87–91] and/or nicotinamide adenine dinucleotide phosphate (NADPH) oxidases, such as NOX4 [92], and can be associated with an influx of calcium via TRPC [93]. With regard to ROS in mediating hypoxic responses, there is current controversy as to whether they are up- or downregulated during hypoxia [94, 95].
Moreover, the voltage-independent two-pore-domain K+ channel, TWIK-related acid-sensitive K+ channel (TASK)-1 is also inhibited by hypoxia, leading to membrane depolarization and calcium entry through L-type channels [96, 97]. Interestingly, it was recently shown that the inhibition of TASK-1 is also mediated by an ET-1-dependent mechanism [98]. In addition to such channels, L-type Ca2+ channels are shown to be a major cellular Ca2+ entry pathway [99, 100], (Fig. 15.2).
All these ion alterations are associated with more contractile, apoptosis-resistant, proliferative, and migratory PASMCs under hypoxic conditions [80–83, 100]. The role of HIF-2α in this context is still not clear.
Another recently discovered key player for the development of hypoxia-induced but also nonhypoxia-induced PH, is Fhl1, a protein known to be involved in muscle development (Fig. 15.1). It could be demonstrated that HIF-1α, as well as HIF-2α, induced Fhl-1 expression not only in different animal models of PH but also in human patients with idiopathic pulmonary arterial (PA) hypertension [101]. The increase in Fhl-1 causes elevated proliferation and migration of PASMCs, contributing to vascular remodeling [101].
15.3.4 Hypoxia-Independent Mechanisms Leading to the Development of COPD and PH
Hypoxia has long been thought to be the primary driving force for the development of PH in COPD supported by studies showing a close relationship between mPAP and/or pulmonary resistance and alveolar hypoxia [12, 102, 103]. However, evidence that the causal factors can be hypoxia-independent and more complex is severalfold. (i) It has been shown that oxygen therapy is not able to fully reverse PH in COPD [46, 52]. (ii) Histological investigation in lungs from COPD patients with PH revealed involvement of all vessel layers characterized by prominent intimal thickening, medial hypertrophy, and muscularization of small arterioles [36, 104], whereas hypoxia-induced vascular remodeling is mainly restricted to the vessel media. This finding is supported by the fact that these pulmonary vascular alterations also occurred in nonhypoxic patients with mild airflow obstruction and smokers without any parenchymal disorder, suggesting that vascular changes may be driven by mechanisms independent of hypoxia/hypoxemia [23]. (iii) Consistent with the findings described in humans, a recent study demonstrated that in mice chronically exposed to tobacco smoke, pulmonary vascular remodeling and PH preceded the development of emphysema, and these changes were independent of hypoxia; there was neither hypoxia in the smoking chamber nor hypoxemia in the mice [20]. In addition, this study showed that the expression of genes involved in important pathways associated with PH and COPD, such as apoptosis, proliferation, oxidative stress , extracellular matrix, and inflammation, followed a completely different pattern compared with that observed in chronic hypoxia-induced vascular remodeling, indicating that the smoke- and hypoxia-driven mechanisms were different [20]. The combination of cigarette smoke and hypoxia can have synergistic effects in terms of affecting the vasculature. Guinea pigs exposed to cigarette smoke and subsequently to hypoxia showed a stronger elevation of pulmonary artery pressure and a more pronounced remodeling in small vessels compared with exposure to only one of these stimuli [105].
Additional animal studies reported a direct effect of cigarette smoke on the parenchyma and vasculature. Cigarette smoke can increase the expression of vasoactive mediators in pulmonary arteries [106], affecting the gene expression in pulmonary arteries [107]. The exposure of guinea pigs to chronic cigarette smoke induced emphysema, which was associated with a reduced lung capillary density [108]. It was reported that cigarette smoke extract (CSE) can induce ET-1 in pulmonary artery ECs [109] and reduce prostacyclin synthase expression [110]. Moreover, CSE is able to induce the production of superoxide in ECs, which in turn reacts with NO to peroxynitrite [111]. This radical can inactivate VEGFR2 signaling [112], which is important for EC maintenance and growth. Of interest, CSE-induced EC apoptosis via p53 [113] can be prevented by the phosphodiesterase (PDE)-5 inhibitor sildenafil [114], which leads to increased levels of cyclic guanosine monophosphate (cGMP), an important second messenger. This finding suggests that the cGMP pathway is involved in this context and decreased after smoke exposure.
15.4 Impairment of the Endothelium: Endothelial Dysfunction
New advances regarding the pathogenesis of PH in COPD suggest that an endothelium-derived vasoconstrictor-dilator imbalance caused by endothelial dysfunction associated with decreased expression or uncoupling of endothelial NO synthase (eNOS) could be a driving force for PH development (Fig. 15.3). In this context, expression of VEGF and serotonin transporters seem to be increased [37, 38, 115, 116]. ECs are important for the regulation of vascular homeostasis [117]. They reduce the vascular tone [118] and regulate pulmonary vessel adaptation to increases in blood flow [119] and hypoxia [120, 121]. Endothelial dysfunction has been reported in patients with end-stage COPD who had undergone lung transplantation [115], as well as in patients with mild-to-moderate COPD [37]. The endothelial function is influenced by the expression of vasoreactive mediators controlling vasoconstriction (also proproliferative for SMCs) or vasodilation (also antiproliferative for SMCs). On the one hand, it has been shown that the vasoconstrictive protein ET-1 was increased in patients with primary and secondary PH, including COPD patients [122] and, on the other hand, vasodilative mediators such as eNOS [123, 124] and prostacyclin synthase (PGI2-S) [110] were shown to be downregulated in pulmonary arteries of patients with severe COPD. CSE and/or acrolein (a potent αβ-unsaturated aldehyde contained in cigarette smoke) can decrease the expression of PGI2-S in human pulmonary artery ECs (HPAECs) [110], supporting the hypothesis that its downregulation in COPD patients arises directly from the cigarette smoke ingredients. After exposure to cigarette smoke for 8 months, mice developed emphysema and PH, as well as concomitant downregulation of eNOS in lungs and vessels, supporting the observation in humans [20]. Of interest, eNOS-deficient mice developed emphysema and PH upon exposure to cigarette smoke, whereas inducible NO synthase (iNOS)-deficient mice did not [20].

Fig. 15.3
Endothelial dysfunction as a causing factor for the development of vascular remodeling. Cigarette smoke and inflammatory mediators can cause endothelial dysfunction which is triggered by a a disbalance of vasodilative and vasoconstrictive molecules towards an excess of vasoconstrictors, and b damage/dysregulation of EC signaling. Additionally, vascular progenitor cells (VPCs) are attracted to the damaged endothelium. Such VPCs can either contribute to repair by differentiation into ECs or to remodeling by differentiation into SMCs. Furthermore, an endothelial-to-mesenchymal transition (EMT) may occur, resulting in an SMC phenotype. Vasoconstriction and altered endothelial cell signaling are stimuli for smooth muscle cells (SMCs) to proliferate, resulting in vascular remodeling, increased pulmonary artery pressure and, finally, pulmonary hypertension
15.5 Inflammatory Cells
Systemic inflammation is a known phenomenon in COPD [125, 126]. Thus, inflammation could also contribute to the pathogenesis of PH in patients with COPD. In fact, the degree of pulmonary vascular remodeling correlates with the amount of inflammatory cells seen in small airways [14, 51]. Compared with nonsmokers, COPD patients show increased numbers of inflammatory cells invading the adventitia of pulmonary muscular arteries [127]. These cells predominantly consist of activated T lymphocytes, especially CD8+ T cells [115, 127], which are increased in the arterial adventitia of smokers with normal lung function. The ratio of CD4+/CD8+ is reduced compared with nonsmokers, and is comparable to the situation in patients with mild-to-moderate COPD [127]. The correlation of IL-6 (an inflammatory mediator) expression and elevations in mPAP supports a possible role of inflammation in the pathogenesis of PH (in COPD) [128].
15.6 Oxidative and Nitrosative Stress and the Influence in Vascular (Patho-)Physiology
Evidences for oxidative stress in COPD patients are numerous [7, 129]. Oxidative stress is the result of an imbalance between oxidants and antioxidants in favor of oxidants. Cigarette smoke itself contains high concentrations of ROS [130]. Elevated concentrations of H2O2 and 8-isoprostane (oxidative stress markers) can be found in exhaled breath condensate of smokers and ex-smokers, as well as during exacerbations [131–133]. ROS can negatively influence the function of antiproteases, such as α1-antitrypsin and SLPI. This negative influence leads to a protease/antiprotease imbalance accelerating the degradation of elastin in the lung parenchyma, resulting in emphysema [134]. In addition, ROS play an important role in the vasculature. Traditionally, macrophages have been considered as the major ROS source, even in vessel walls [135]. However, several studies showed that all vascular cells (endothelial, smooth muscle, adventitial cells) produce ROS in different amounts, depending on the stimuli to modulate cellular function [136].
ROS, for example produced by macrophages, are normally involved in the elimination of pathogens and as such are critical to the organism. ROS and reactive nitrogen species (RNS) are able to cause protein modification and DNA damage [137]. Indeed, NO, generated by NO synthases, reacts with superoxide (O2•–) to form the potent oxidant peroxynitrite (ONOO) [138]. This peroxynitrite can react with tyrosine residues from proteins to form nitrotyrosine, shown to be increased in COPD patients [47, 48]. In the vasculature, ROS play an important physiological role, participating as second messengers in endothelial function, in smooth muscle and EC growth and survival, and in the process of remodeling of the vessel wall [139]. Under pathophysiological conditions, these responses are uncontrolled and imbalanced, respectively [140–142]. The main vascular ROS is the superoxide anion, which can inactivate NO, as mentioned above, by generating peroxynitrite. Since NO is an important vasodilator, the reaction with ROS influences the vascular tone [143, 144]. Superoxide can be converted to H2O2 by superoxide dismutase (SOD), which is a more stable ROS. The depletion of H2O2 is carried out by catalase and glutathione peroxidase producing H2O. Hydrogen peroxide and other peroxides regulate growth-related signaling in vascular SMCs and inflammatory responses in vascular lesions [141, 145]. ROS can activate several vascular signaling cascades, such as ERKs and MAPKs, which are important in cell growth, proliferation, apoptosis, and differentiation. Furthermore, receptor and nonreceptor tyrosine kinases (shown to be involved in cardiovascular remodeling and damage), and protein tyrosine phosphatases and transcription factors such as NF-κB and AP-1, which induce vascular inflammation [146, 147], are affected by ROS. Several studies reported the role of ROS in growth processes contributing to vascular remodeling and injury. Angiotensin II can lead to NADPH oxidase-generated superoxide production, mediated by the angiotensin type I (ATI) receptor, which is converted to H2O2 by SOD acting as a second messenger that results in hypertrophy or hyperplasia of vascular SMCs [148, 149]. This angiotensin II-induced reaction can be inhibited by the flavoprotein inhibitor diphenyleneiodonium (DPI) [148], catalase [149], and knockdown of p22phox [150], supporting the involvement of NADPH oxidases in the vasculature.
ROS are also able to promote vascular remodeling by increasing deposition of extracellular matrix proteins . Collagen and elastic fibers can be degraded by proteinases, especially matrix metalloproteinases (MMPs). These MMPs are secreted by macrophages and vascular SMCs in an inactive form [151]. ROS, for example peroxynitrite, activate MMP2 and 9 in human SMCs, leading to degradation of the basement membrane and elastin [152]. Use of a hypertension model of aldosterone-induced systemic oxidative stress revealed that ET-1-associated processes lead to vascular remodeling [153]. Redox-sensitive inflammatory processes can also contribute to vascular remodeling. Expression of the inflammation-related intracellular adhesion molecule-1 (ICAM-1) is elevated in the aorta from aldosterone-treated rats [153]. Furthermore, Liu et al. [154] reported that angiotensin II-induced oxidative stress caused tissue hypertrophy which was associated with increased ICAM-1 expression. Of interest, NADPH oxidases were involved in this context, producing ROS [154]. It is of note that not only the vascular but also the phagocytic NADPH oxidase is involved in superoxide production in cardiovascular diseases because monocytes and lymphocytes can infiltrate cardiovascular tissues [135].
Taken together, elevated occurrence of superoxide, H2O2, and reduced NO bioavailability by reaction with superoxide forming peroxynitrite can contribute fundamentally to vascular remodeling and emphysema development.
15.7 Recent Advances in Molecular Mechanisms of COPD Associated with Vascular Remodeling/PH
15.7.1 Inducible Nitric Oxide Synthase as a Key Player for the Development of Cigarette Smoke-Induced PH and Emphysema
A recent study identified the major NO source in the context of smoke-induced emphysema and PH in mice [20]. Cigarette smoke led to an upregulation of the iNOS mRNA and protein expression, predominantly in small pulmonary vessels, and was associated with increased NO generation. Interestingly, eNOS was downregulated when the disease was established, in mice as well as in COPD patients . It is most probable that the vasodilative NO effect did not appear because of the simultaneous abundance of ROS from both external (cigarette smoke) and internal sources. This study suggested that the subsequent formation of peroxynitrite , most probably increased in this context, had proapoptotic effects (as well as antiproliferative effects) on alveolar epithelial cells type II (AECII) and ECs, promoting emphysema development, vessel loss, and vascular remodeling. Moreover, the level of nitrotyrosine was increased in those mice as well as in smokers without COPD and patients with severe COPD. It is likely that the downregulation of eNOS was associated with uncoupling of this enzyme, leading to the switch of NO to superoxide production, which increased the oxidative stress . Mice deficient in iNOS but not eNOS were protected from vascular remodeling, PH, and emphysema. Treatment with an iNOS inhibitor (L-NIL) could not only prevent disease development but also promote lung regeneration in mice previously exposed to cigarette smoke for 8 months.
Moreover, it has been shown that the development of PH was mediated by iNOS-carrying bone-marrow-derived cells, whereas emphysema development was dependent on iNOS in nonbone-marrow-derived cells [20] (Fig. 15.4). This phenomenon shows that PH and emphysema can develop independently of each other, and could also explain the discrepancy that not all COPD patients also suffer from PH, if such results are transferable to the human situation.

Fig. 15.4
Identification of inducible nitric oxide synthase (iNOS) as an essential factor for the development of cigarette smoke-induced emphysema and pulmonary hypertension (PH) in mice. Cigarette smoke-mediated upregulation of iNOS leads to excessive NO production. The formation of peroxynitrite, resulting from the reaction of NO with superoxide, was suggested to mediate emphysema and PH development. Superoxide can derive from cigarette smoke itself and/or from uncoupled endothelial NOS (eNOS), nicotinamide adenine dinucleotide phosphate (NADPH) oxidases, xanthine oxidases, cyclo- and lipooxidases, and mitochondria. Of interest, iNOS generated by nonbone-marrow-derived cells (NBMDC), possibly vascular cells, leads to lung destruction, resulting in emphysema, whereas elevated iNOS expression in bone-marrow-derived cells (BMDC) causes vascular remodeling. Treatment with the specific iNOS inhibitor L-NIL prevents or even reverses pathological alterations
Quite recently, it could be demonstrated in mice and guinea pigs that stimulation of the soluble guanylate cyclase (sGC) could not only prevent the cigarette smoke-induced development of vascular remodeling but also emphysema [155]. The sGC is an enzyme that uses iNOS-generated NO to produce cGMP from guanosine triphosphate (GTP). cGMP acts as a second messenger, amongst others mediating vasodilation, which can decrease the vascular pressure. The combination of the iNOS-related findings [20] with this study demonstrates the important role of the NO–sGC–cGMP axis for the physiology and pathophysiology of the pulmonary vasculature. It has already been suggested that dysregulation of this axis contributes to pulmonary diseases and PH [156–158]. In line with previous findings [20], prevention from vascular remodeling was associated with prevention from emphysema, although causality was not investigated in these studies.
15.7.2 Reactive Oxygen Species as a Trigger for Vascular Remodeling
Although cigarette smoke itself produces ROS, causing oxidative stress , the additional internal ROS sources produced by the organism are still not fully resolved. Depending on the circumstances, several sources such as NADPH oxidases, mitochondria, xanthine oxidase, cyclooxygenases, lipooxygenases, and uncoupled eNOS come into consideration. As mentioned before, uncoupled eNOS can contribute to oxidative stress in terms of COPD [159–163]. Based on experimental and clinical studies, NADPH oxidases are suggested to be the predominant superoxide-producing enzymes in the context of oxidative stress in cardiovascular diseases [164]. The classical NADPH oxidase is expressed in phagocytes; it produces O2•– as a defense mechanism against bacterial infections [135]. The intrinsic enzyme consists of membrane-bound catalytic subunits that produce the ROS and cytosolic subunits which regulate the function of the enzyme. In phagocytes gp91phox (= NOX2; ‘phox’ = phagocytic oxidase) is associated with p22phox, being essential for its function [165], and both are located in the membrane. They form a complex with the cytosolic subunits p40phox, p47phox, and p67phox, as well as the G-protein Rac2 [166]. All these subunits, as well as the other membrane-bound enzymes NOX1, NOX4, and NOX5, and homologs to p47phox (NOXO1) and p67phox (NOXA1), are expressed in vascular cells. NOX5 seems to be active without any regulatory subunit. NOX4 is constitutively active, is associated with p22phox, and its function appears to be independent of regulatory subunits, in contrast to NOX1 and NOX2, which need to be activated [167]. The activity of NOX1 is predominantly regulated by NOXO1 and NOXA1 [168]. Activation by p47phox and p67phox is also possible but is less effective than involvement of NOXO1 and NOXA1 [168, 169]. In the case of NOX2, p47phox is phosphorylated upon cell stimulation, followed by complex formation with the other cytosolic subunits, mediating migration to the membrane-bound subunit. Electrons are then transferred from the substrate NADPH to O2, resulting in superoxide (O2•–) production [170].
In addition to inflammatory processes often related to immune defense or cigarette smoking, NADPH oxidases can be stimulated by many factors in cardiopulmonary vascular diseases. Vasoactive agonists such as angiotensin II, ET-1, and tumor necrosis factor-α (TNFα) can regulate NADPH oxidases in vascular cells [153, 154, 171]. Even genetic factors might be involved in NADPH oxidase-dependent superoxide production. For instance, polymorphisms in the promoter region of the gene encoding p22phox have been identified. Such polymorphisms can increase the activity of the promoter in vascular SMCs [172], which were shown to be associated with essential hypertension [173] and decreased NO bioavailability [174].
In terms of remodeling, ROS can influence several intracellular signaling cascades, e.g. activation of ERKs and MAPKs, which affect cell growth and differentiation; protein tyrosine phosphatases and transcription factors, for instance NF-κB and AP-1, inducing proinflammatory genes associated with hypertension and atherosclerosis ; and receptor and nonreceptor tyrosine kinases, which have been shown to be involved in cardiovascular remodeling and vascular damage [146].
Because of the fact that monocytes and lymphocytes are able to infiltrate cardiovascular tissues and pulmonary vessels, it is important to note that in cardiovascular diseases, such as COPD, not only vascular NADPH oxidase can contribute to ROS formation, and hence to vascular remodeling, but phagocytic oxidase can also contribute [175].
15.7.3 Neprilysin Downregulation Provokes Pulmonary Vascular Remodeling
A recent study demonstrated that neprilysin (neutral endopeptidase [NEP]) might be an important factor for the susceptibility of humans to pulmonary vascular remodeling in response to smoke inhalation and hypoxia [176] (Fig. 15.5). NEP is a transmembrane zinc peptidase that is widely expressed, including in PASMCs, PAECs, and fibroblasts [177]. Its activity and expression is decreased by cigarette smoke [178], hypoxia [179, 180], and ROS [181]; it is particularly important in vascular SMCs in terms of remodeling [176]. A genetic depletion of NEP in mice resulted in increased severity of PH associated with stronger proliferation of PASMCs compared with wild-type mice. This finding suggests a protective role of NEP against PH, partly by suppressing proliferation and migration of PASMCs [180].

Fig. 15.5
Scheme of proposed neprolysin (NEP)-dependent mechanisms leading to vascular remodeling. Extracellular stimuli, such as hypoxia and cigarette smoke, activate pathways in vascular cells, causing downregulation of NEP expression and/or activity which was seen in patients with COPD associated with pulmonary hypertension (PH). Stimuli can have direct negative effects on NEP, and also indirectly by 1) increasing reactive oxygen species (ROS) and platelet-derived growth factor (PDGF); and 2) activation of other pathways, in part receptor-mediated. NEP downregulation leads to elevated proliferation, migration, inflammation, angiogenesis, and vasoconstriction mediated, amongst others, by depicted molecules, causing vascular remodeling. The ROS effect on NEP can be inhibited by the superoxide dismutase (SOD) mimetic tiron. Colored boxes indicate involvement in the respective pathway; red arrows represent inhibition/downregulation; green arrows represent activation/upregulation. ET-1 endothelin-1, FGF-2 fibroblast growth factor, AT-II angiotension-II, PASMC pulmonary artery smooth muscle cells, EC endothelial cells, FB fibroblasts
Interestingly, Wick et al. [176] showed that NEP expression was decreased in lungs from COPD patients with PH and non-COPD PH patients, especially in the distal vasculature where prominent remodeling occurs. NEP is involved in many peptidase-dependent (e.g. degradation of vasoactive neuropeptides) and -independent (e.g. interaction of signaling molecules with NEP’s intracellular cytosolic subunit) signaling pathways [182, 183], but its endopeptidase activity-dependent pathways influencing vascular remodeling are not yet well understood. Wick et al. postulated that increased proliferation/migration of dedifferentiated SMCs or myofibroblasts may promote pulmonary vascular remodeling and PH if NEP is less active or downregulated, perhaps mediated by platelet-derived growth factor (PDGF) whose expression is inversely correlated to NEP . Recent data from Karoor et al. support this assumption. They showed that PDGF receptor (PDGFR) signaling was constitutively active in NEP−/− cells and lungs; this effect could be attenuated by the endothelin A (ETA) receptor antagonist ambrisentan [184]. Additionally, a loss of NEP could have inflammatory, angiogenic, and vasoconstrictive effects on vascular cells. The anti-inflammatory action of NEP could be explained by NEP-dependent degradation of the proinflammatory substrates substance P and bradikinin. The decrease in NEP after cigarette smoke and hypoxia may also enhance the angiogenic effect of fibroblast growth factor (FGF)-2 [182], as well as the proproliferative and vasoconstrictive properties of ET-1 [185] and bombesin-like peptides [186], all being substrates of NEP.
Recently, it was demonstrated in PASMCs that FGF-2 and ET-1 synergize with PDGF in increasing phosphorylation of Src kinase (amongst others activating PDGFR) and PDGFR, which promoted migration and proliferation of the cells [184]. The phosphatase PTEN (phosphatase and tensin homolog) also plays an important role in vascular biology. The loss of PTEN results in PH [187]. It is inactivated by phosphorylation (mediated by Src and PDGFR) and downregulated in NEP-deficient PASMCs. This downregulation could be rescued by NEP overexpression in NEP null cells or by downregulation of Src or PDGFR by small interfering RNA (siRNA). These observations suggest that NEP-dependent mechanisms protect against the inactivation of PTEN [184]. Moreover, NEP can be inactivated by ROS, as shown by decreased activity in the presence of H2O2 and improved NEP activity when an antioxidant, the SOD mimetic tiron, was present [176].
Of interest, early studies suggest that inhibition of NEP could be beneficial for the treatment of PH [188, 189]. This proposal is based on the fact that NEP can inactivate atrial/brain natriuretic peptides (ANP/BNP), which promote vasodilation by increasing cGMP mediated via natriuretic peptide receptor-A (NPR-A) [190]. cGMP-dependent protein kinase (PKG), cGMP binding PDEs, and cyclic nucleotide-gated ion channels bind cGMP, in which PKG seems to be the main mediator of cGMP signals [191–193]. Binding of ANP/BNP-induced cGMP activates PKG, leading to the catalytic transfer of phosphate from ATP to target proteins. These phosphorylated proteins translate the extracellular stimuli into specific biological outputs [194], such as vasodilation.
It has been shown that NEP antagonists, alone and in combination with angiotensin-converting enzyme (ACE) and endothelin-converting enzyme (ECE) inhibitors, were able to improve cardiac function, decrease systemic blood pressure, and limit cardiac hypertrophy [195–198]. Nevertheless, side effects were observed if single NEP inhibitors or dual inhibitors (NEP/ACE or NEP/ECE) were used. Nowadays, even triple vasopeptidase inhibitors (NEP/ACE/ECE) are under investigation, with promising preliminary results showing fewer side effects; in particular, the increase in ET-1 can be reversed by simultaneous application of an ECE inhibitor [196]. These combination therapies and the different mechanisms of NEP , ACE, and ECE inhibition have been extensively reviewed by Daull et al. [196].
However, recent observations support the possibility that NEP could be protective against PH [176, 180, 199]. One explanation for the discrepancy between the beneficial cardiac effects and harmful pulmonary effects of NEP inhibition is the well-known phenomenon that the pulmonary and systemic circulations usually respond to hypoxia (a major stimulus for PH) in opposite ways: pulmonary vessels contract to redirect blood flow to better oxygenated areas of the lung, whereas systemic vessels dilate to increase the flow of oxygenated blood to areas of tissue hypoxia or ischemia [180].
In conclusion, in terms of the lung, it is suggested to increase NEP to treat PH, whereas cardiac NEP inhibition could be used for treatment of hypertrophy and improvement of cardiac function. This dichotomy clearly demonstrates that a possible treatment must be dependent not only on the target itself but also on the localization of the target. These data should be kept in mind when considering NEP-related drug therapies.
15.8 Conclusions
The mechanisms of COPD and PH in COPD are still far from being fully understood. Studies primarily from the last decade have shown that vascular remodeling and PH can occur in COPD, not only in severe cases but also in mild-to-moderate forms of the disease, or even in smokers without airflow limitations. Investigations of the molecular mechanisms of COPD and the occurrence of pulmonary vascular remodeling established that COPD associated with PH and pulmonary vascular remodeling is a complicated multifactorial disease involving hypoxia-related and hypoxia-unrelated mechanisms, inflammation, and endothelial dysfunction . Recent advances have changed the long-standing view of the pathobiology of COPD. In the past, vascular alterations (vascular remodeling and PH) have been seen to be secondary events occurring after destruction of the parenchyma, predominantly caused by hypoxia/hypoxemia. However, recent studies have demonstrated that such vascular abnormalities can be early events in COPD, preceding airflow limitations and emphysema, and can be independent of hypoxia. It has been shown, at least in mice, that PH and lung emphysema triggered by tobacco smoke can occur independently, and suggest that vascular molecular alterations can be a trigger for lung emphysema development.
Further elucidation of the contribution of pulmonary vascular changes to COPD development may help to identify new therapeutic concepts for this disease.
References
1.
Agusti AG, Noguera A, Sauleda J, Sala E, Pons J, Busquets X. Systemic effects of chronic obstructive pulmonary disease. Eur Respir J. 2003;21(2):347–60.PubMed
2.
Sertogullarindan B, Gumrukcuoglu HA, Sezgi C, Akil MA. Frequency of pulmonary hypertension in patients with COPD due to biomass smoke and tobacco smoke. Int J Med Sci. 2012;9(6):406–12.PubMedCentralPubMed
3.
Black PN, Ching PS, Beaumont B, Ranasinghe S, Taylor G, Merrilees MJ. Changes in elastic fibres in the small airways and alveoli in COPD. Eur Respir J. 2008;31(5):998–1004.PubMed
4.
Barnes PJ. Chronic obstructive pulmonary disease. N Engl J Med. 2000;343(4):269–80.PubMed
5.
Sharafkhaneh A, Hanania NA, Kim V. Pathogenesis of emphysema: from the bench to the bedside. Proc Am Thorac Soc. 2008;5(4):475–7.PubMedCentralPubMed
6.
Churg A, Cosio M, Wright JL. Mechanisms of cigarette smoke-induced COPD: insights from animal models. Am J Physiol Lung Cell Mol Physiol. 2008;294(4):L612–31.PubMed
7.
Repine JE, Bast A, Lankhorst I. Oxidative stress in chronic obstructive pulmonary disease. Oxidative Stress Study Group. Am J Respir Crit Care Med. 1997;156(2 Pt 1):341–57.PubMed
8.
Barbera JA, Peinado VI, Santos S. Pulmonary hypertension in chronic obstructive pulmonary disease. Eur Respir J. 2003;21(5):892–905.PubMed
9.
Peinado VI, Pizarro S, Barbera JA. Pulmonary vascular involvement in COPD. Chest. 2008;134(4):808–14.PubMed
10.
Wright JL, Levy RD, Churg A. Pulmonary hypertension in chronic obstructive pulmonary disease: current theories of pathogenesis and their implications for treatment. Thorax. 2005;60(7):605–9.PubMedCentralPubMed
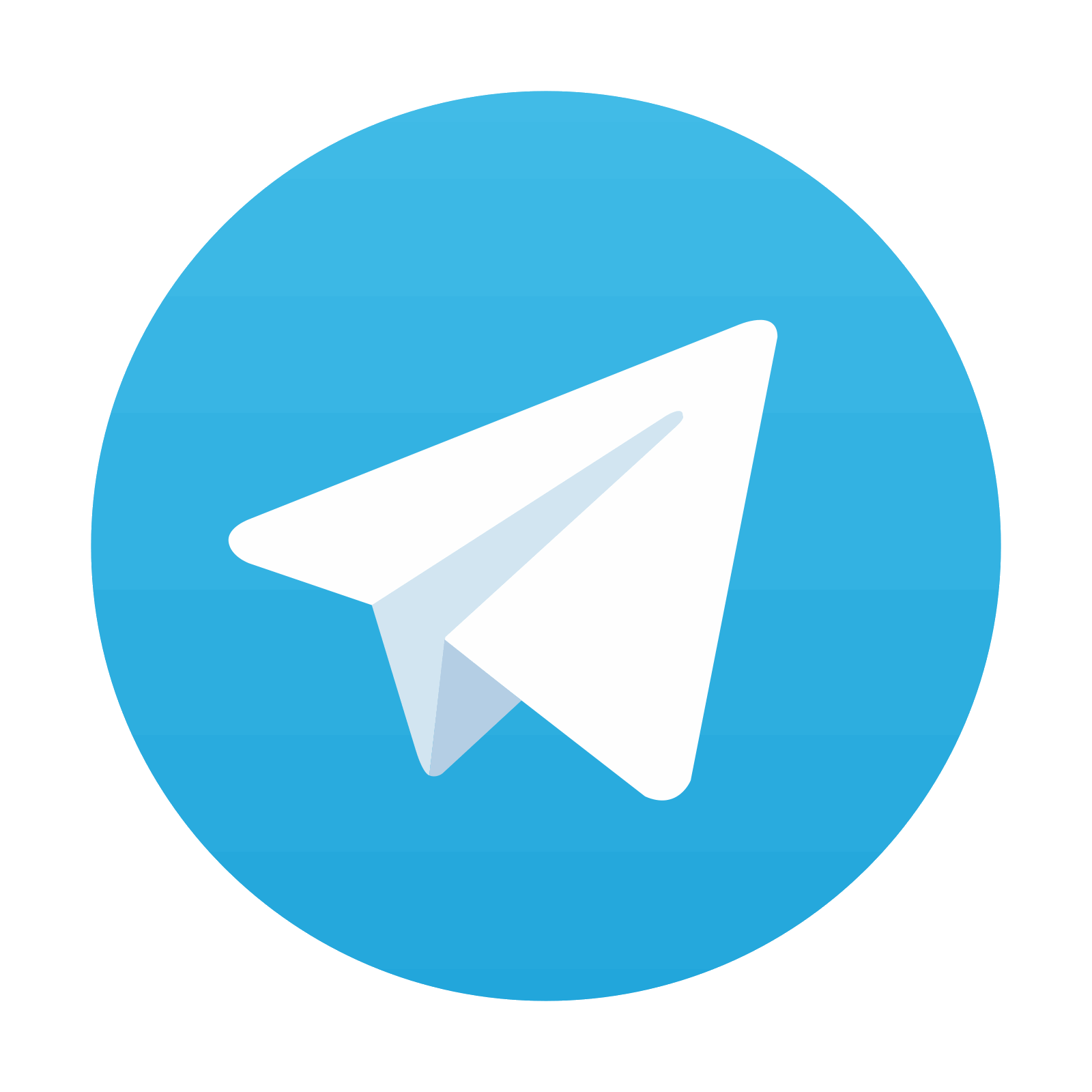
Stay updated, free articles. Join our Telegram channel
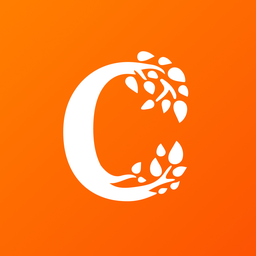
Full access? Get Clinical Tree
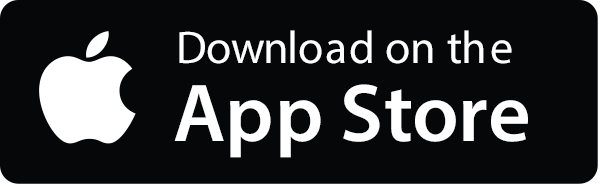
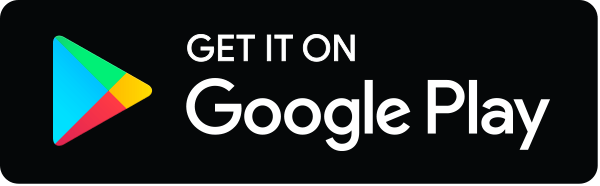