CHAPTER 4 The Origins of Behavior and Cognition in the Developing Brain*
Pediatricians specializing in developmental, learning, and behavioral problems have a strong interest in how the brain develops. As clinicians, pediatricians are also interested in the related topic of neural plasticity, especially how development can go pathologically “off track” and how treatment can help correct its course. We have argued1,2 that brain development can be described as a complex scaffolding of three categories of neural processes: gene-driven, experience-expectant, and experience-dependent. Gene-driven processes, which are comparatively insensitive to experience, serve to guide the migration of neurons, to target many of their synaptic connections, and to determine their differentiated functions. Experience-expectant processes correspond approximately to “sensitive periods,” developmentally timed periods of neural plasticity for which certain types of predictable experience are expected to be present for all juvenile members of a species. Not all brain development, however, is determined by gene-driven processes. Some species have a survival advantage if they can adapt to the environment or incorporate information from it. Indeed, many mammalian species have evolved specialized structures that can incorporate massive amounts of information. Because they have a long evolutionary history, the specialized systems vary across species and occur in multiple brain regions, so that there is no single “place” or “process” for learning and memory. Some types of neural plasticity have evolved to be incorporated into the developmental schedule of brain development, whereas others have evolved to serve the individual’s needs by incorporating information unique to their environment. This type of neural plasticity is termed experience-dependent, and it corresponds approximately to ordinary learning and memory: that is, encoding information that has adaptive value to an individual but is unpredictable in its timing or nature.
We emphasize a contemporary model of brain development that is derived from the study of dynamic, nonlinear systems. The dynamic systems perspective suggests that individuals use the interaction of genetic constraints and environmental information to self-organize highly complex systems (especially brains). Each organism follows a potentially unique and partly self-determined developmental path of brain assembly to the extent that the organism has unique experiences. The genetically determined restrictions (e.g., the initial cortical architecture) serve as constraints to the system, allowing the interaction of environmental information with existing neural structures to substantially organize and refine neural connections. In this chapter, which extends and amplifies earlier work by Black and colleagues,3 we review the evidence for these three processes, integrate them into a general model of brain development, provide evidence that the human brain is similarly plastic, and then apply this information to issues of children’s development and behavior.
GENE-DRIVEN PROCESSES
Gene-driven processes provide much of the basic structure of the brain and are intrinsically resistant to experience. Waddington4 described this tendency to resist deviations from predetermined pathways of development as canalization. Some of these genetically determined structures have evolved to constrain and organize experiential information, facilitating its storage in the brain in massive quantities. We now know much of the molecular biological processes of cell differentiation, neuron migration, and cell regulation and signaling. These processes are capable of building enormously complex neural structures without substantial input from the external environment. Evidence for the importance of gene-driven processes can be found in the tens of thousands of genes uniquely expressed in rat brain development.5,6 Indeed, in order to protect brain development, much of the basic organization of most nervous systems is largely impervious to experience. Neural activity that is intrinsically driven, such as that arising from the retina in utero, can play a role in these organization processes, by means of some of the mechanisms that seem also to be used later in the encoding of experience. For example, myelination of the optic nerve appears to be initially driven by spontaneous retinal activity and subsequently influenced by visually generated stimulation of the retina.7–10 Astrocytic development is also influenced by activity11 and is discussed in more detail later in this chapter. This theme of molecules and mechanisms, borrowed for other purposes in brain development or plasticity, can be found many times in this chapter.
TISSUE INDUCTION AND FORMING THE BASIC BRAIN PATTERN
Early central nervous system (CNS) development involves an ordered sequence of processes, beginning with formation of the neural plate and followed by an orderly program of further inductions.12 As in many embryological processes, brain tissue induction typically involves an organizer and its developmental target. Neural induction is familiar to physicians from the embryological process of gastrulation, in which the neuroectoderm just organizes itself. The signaling factors include activation of receptor tyrosine kinases, insulin-like growth factors and fibroblast growth factors, controlled inhibition of other signaling pathways (e.g., Noggin and Chordin), and the wingless pathway.13 A region of the neuroectoderm becomes differentiated by these signals, and its lateral edges become the neural crest and, later, the peripheral nervous system. Thus, these early signals point the tissue toward neural development or toward other ectodermal development. Many molecular mechanisms of neuronal development and organization have been preserved across species and time, in such a way that they remain remarkably similar in species as diverse as the fruit fly, amphibian, and mouse.14 We contend that some of these same mechanisms are then exploited later in development for analogous functions in critical periods and in learning and memory.
Some of the most important and unique characteristics of human brain structure appear to have evolved from relatively simple adjustments of genes controlling neuron number, modifying the rate of brain development, and regulating brain plasticity.15 Within the neural ectoderm, the spatial pattern determines much of future brain anatomy.16 The signals further differentiate the neural tube along an anteroposterior dimension and a mediolateral axis. After this point, each compartment has its own program of differentiation. The anteroposterior segments differentiate into the rhombencephalon (hindbrain), mesencephalon (midbrain), and prosencephalon (forebrain). Each of these subdivisions then follows a genetically controlled program of cell division and migration to swell into rhombomeres in the hindbrain and prosomeres in the forebrain, each of which becomes an important neural structure in the mature brain. Of course, early brain morphogenesis and control are very similar for many vertebrates. In contrast, analysis of genetic drift shows that the abnormal spindle-like microcephaly-associated (ASPM) gene, which affects overall brain size, began changing only in the past 5 million to 6 million years of human evolution to allow larger brain size.17 This recent adaptive evolution in a gene controlling brain growth is consistent with the role of key, distinctive features in human brain development: the timing of maturation and regulation of size, connectivity and plasticity.
The mediolateral regionalization produces distinct tissues that are longitudinally aligned along the long axis of the CNS. Medial inductions are regulated by substances produced by axial mesodermal organizers: the notochord and the prechordal plate. These organizers are midline structures that lie underneath and produce substances such as sonic hedgehog that induce the medial neural plate to form the floor plate and basal plate. Growth factor proteins such as transforming growth factor–β mediate inductions from the lateral edge of the neural plate that are produced by the nonneural ectoderm. Lateral inductions are likely to be essential for the development of the neural crest, alar plate, and roof plate. Further patterning is determined in a checkerboard organization of brain subdivisions specified by the coordinates of anteroposterior and mediolateral location. Within the checkerboard, specific cues trigger the formation of swellings and vesicles that later become the telencephalon, eyes, and posterior pituitary gland. Although the process of regionalization subdivides the neural plate into the major brain structures, the process of morphogenesis transforms the shape of the neural plate into first a tube and then a complex tube with flexures and evaginations. As the neural plate transforms into the neural tube (neurulation), it converts the lateral-medial dimension of the neural plate into the dorsal-ventral dimension of the neural tube.14 The fusing of the neural tube is complete 26 days after conception in humans.18 The neural tube now has four ventral-to-dorsal subdivisions—the floor, basal, alar, and roof plates—each of which extends along much of the anteroposterior axis of the CNS and contributes to the distinct functional elements of the nervous system. The basal plate is the origin of the motor neurons, the alar plate is the origin of the secondary sensory neurons, and the floor plate is devoid of neurons and has several functions that are required during development. Like the notochord, the floor plate produces sonic hedgehog and is believed to serve as a secondary organizer guiding certain sensory neurons. Most of the roof plate forms the nonneuronal dorsal midline, including the choroid plexus and the pineal gland (Fig. 4-1).
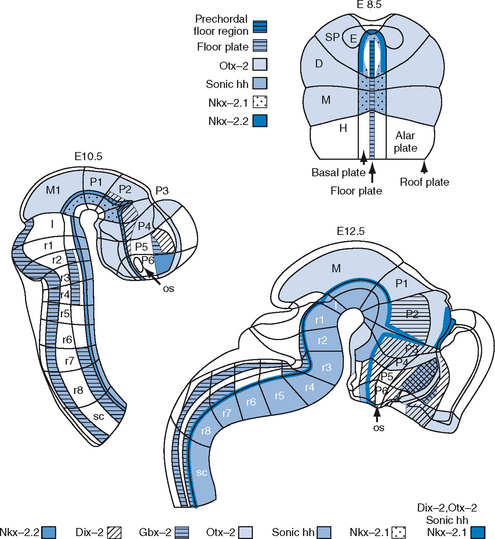
FIGURE 4-1 Six genes are expressed in different regions of the neural plate (E8.5), and neural tube (E10.5, E12.5) in the developing mouse brain. D, diencephalon; E, eyes, H, rhombencephalon-hindbrain; I, isthmus; M, mesencephalon-midbrain; os, optic stalk; p, prosomere; r, rhombomere; sc, spinal cord; SP, secondary prosencephalon.
(From Lumsden A. Krumlauf R. Patterning the vertebrate neuraxis. Science 274:1109–1115, 1996.)
HISTOGENESIS, MIGRATION, AND CELL FATE
As the neural tube organizes into regions that will become major structures (e.g., cerebrum, striatum, thalamus, cerebellum), tissue-specific genetic programs of histogenesis are begun within each region. Histogenesis can be subdivided into two general parts: neuron proliferation and differentiation.19 In general, each of these processes takes place in distinct zones within the wall of the neural tube. Proliferation takes place in the ventricular zone, which lines the inner surface of the neural tube and is adjacent to the ventricular cavity, whereas differentiation takes place largely in the mantle, which surrounds the ventricular zone. The ventricular zone cells are undifferentiated and mitotically active. Each brain region has distinct proliferation programs that regulate the rate of cell division, the number of cell divisions, and the character of cell division. Cell division can be symmetrical or asymmetrical. Symmetrical division produces cells that are identical; both the daughter cells either continue to proliferate or go on to differentiate. Asymmetrical division produces one daughter cell that differentiates and one that continues to proliferate. The regulation of these processes is integral to controlling how many cells are produced and when they are made, and local differences in replication rate give rise to the gross morphological structures of the forebrain, including the massive cerebral cortex.20
Like the ventricular zone, the subventricular zone is involved in the proliferation of brain cells, but it emerges somewhat later, between 8 to 10 weeks of gestation.21,22 In the human, migration into the telencephalic region (destined to become the cerebral cortex, hippocampus, and associated structures) begins at approximately 8 weeks of gestation, when the progenitor cells engage in asymmetrical proliferation to create postmitotic neurons.23 Proliferation ends at approximately 4.5 months of gestation, and the last cells begin their migration.23 Two waves of neurons migrate, in such a way that postmitotic neurons from the ventricular zone are first to leave, and the neurons from the subventricular zone emerge next.24 Cortical neurons migrate in an inside-out pattern, whereby neurons that developed earlier migrate to lower cortical layers, and the cells that developed later travel through and beyond previously migrated neurons for destinations in the outer cortex.25 Therefore, neurons generated in the ventricular zone take up residence in the lower layers of the cortex, and neurons that are derived from the subventricular zone become located in the outer regions of the cortex.
Although neuroanatomy texts may display a dazzling array of brain cell types, all these cells belong to only two major cell classes: neurons and glia.26 There are two major types of neurons: projection neurons, whose axons migrate to distant territories, and local circuit neurons (interneurons), whose processes ramify nearby. There are many distinct types of projection and local circuit neurons. There are two types of CNS-derived glia: astrocytes and oligodendrocytes. Astrocytes, which are believed to be derived from radial glia, probably regulate the local chemical milieu and have been shown in some cases to release neurotransmitters such as glutamate in ways that can affect neuronal activity. Oligodendrocytes produce the myelin sheaths that surround many axons; these sheaths function as insulators that increase the velocity of action potentials. As described later in the chapter, myelin appears to play key roles in regulating the plastic capacities of axons of neurons and the offset of sensitive periods for experiential organization of neuronal networks.27 Thus, these CNS-derived glial cells are increasingly seen as partners of neurons in plasticity during development and neural repair. (Another major glial type, the microglia, is derived from mesoderm and performs a phagocytic and immune system role.) Early in development, the ventricular zone contains proliferative cells that have the potential to produce both neurons and glia. In general, neurogenesis precedes gliogenesis. Most regions of the CNS can produce both neurons and astrocytic glia. Different types of neurons are generated at distinct dorsal-ventral positions in the CNS. Motor neurons in the spinal cord, for example, are generated by ventral progenitors, whereas sensory neurons are generated by dorsal progenitors. Likewise, in the telencephalon, ventral progenitors produce the motor neurons of the basal ganglia, whereas dorsal progenitors produce sensory cortical neurons.
In addition to patterning the regions of the nervous system (e.g., cerebral cortex and basal ganglia), histogenesis also regulates where cells travel (migration) and their ultimate functional fate (differentiation). The mechanisms underlying cell fate decisions in the nervous system involve both intrinsic and extrinsic signals. These signals have integral roles in regulating whether these cells continue to divide, whether they undergo symmetrical or asymmetrical division, and what lineage they will follow. Notch signaling is an example of molecular genetic control of differentiation and is mediated by Notch receptors and their ligands.28 Activation of Notch by its ligand biases a cell not to differentiate; thus, neurogenesis requires inhibition of Notch signaling.29 Notch signaling can control the rate and timing of neuron production, or it can bias progenitors toward an astrocytic fate. Notch signaling activates a complex cascade of molecular switches that culminates in altered gene expression in the differentiating cell.30 Many other types of transcription factors have important roles and serve as examples of gene-driven brain development, including the homeobox, helix-loop-helix, T-box, Winged-helix, and HMG-box families. Each of these families consists of subfamilies; for instance, key homeobox genes include Dlx, Emx, Kx, Otx, Pax, and POU, which control such processes as regional fate, cell type identity, neuronal maturation, and cell migration.16
Once neurons are generated, the next step in their differentiation is migration to the appropriate destination. Each brain region has a specific migration program. In some structures (e.g., cerebral cortex and superior colliculus), migrations are orchestrated to form layered or laminar structures. In most subcortical regions, migrations originate from nuclear structures that generally are not laminar. There are two general types of migration: radial and tangential. Radial migration is movement perpendicular to the wall of the ventricle toward the pial surface; tangential migration is movement parallel to the plane of the ventricle. Radial migration involves the interaction between elongated processes of radial glial cells and migrating immature neurons. Immature neurons are programmed to migrate to a specific location within the wall of the neural tube, where they disengage from the radial glial cell and continue to differentiate. One of the key molecules in regulating this process was identified through the analysis of the reeler mutant mouse, whose reeling behavior reflected the effects of its mutation on functional brain organization.31 In the cerebral cortex of reeler mice, later born neurons fail to migrate past their earlier born counterparts, leading to partial inversion of the usual inside out lamination. The reeler gene encodes a large secreted molecule named Reelin that appears to promote dissociation of neuroblasts from radial glia. Mouse genetic studies have implicated two low-density lipoproteins (VLDLR and ApoER2) as the receptors for the Reelin molecule. Intriguingly, this pathway appears to be significantly disturbed in the neurodevelopmental disorder of schizophrenia.32 Tangential migration of neurons has long been known to occur in the cerebellum and in the rostral migratory stream of the olfactory bulbs. Within the telencephalon, many of the γ-amino butyric acid (GABA)–based local circuit neurons are like cousins rather than like siblings, as they appear to have migrated tangentially from the basal ganglia primordial to the cerebral cortex and hippocampus.19 Progress has also been made in identifying genes that control cytoskeletal processes that are essential for migration. Several of these genes were first identified as causing neuronal migration defects in humans, including lissencephaly-1, doublecortin, and filamin.33 It is of clinical interest that a gene (DCDC2) associated with doublecortin has now been associated with heritable reading disabilities.34
NEURAL PATHWAYS AND SYNAPTIC CONNECTIONS
As the immature neurons and glia migrate from the proliferative ventricular zone to the mantle, they elaborate into more complex cellular structures. Neurons extend thin processes away from their cell body, including multiple dendrites and a single axon that can sometimes traverse long distances to find its targets. (For review, see Tessier-Lavigne and Goodman35 and Grunwald and Klein.36) The growing tip of the axon is called the growth cone. This dynamic weblike structure contains filopodia that extend and retract in multiple directions, seeking potential targets. Certain molecules can attract or repel the growing axons through their specific receptor interactions, whereas other molecules provide paths for the growing axons. Other signals provide more specific information about local branching geometry and pathways. For example, glial cells serve as guideposts for axons. Through fasciculation, late-arriving axons adhere and are bundled together with earlier axons. Molecules on the surface of the axons, some of which are related to immunoglobulins, regulate the pattern of fasciculation and later defasciculation, when the bundle separates.
As axons grow and navigate, they express receptors for guidance molecules that are expressed by neighboring cells.35,37 These processes operate as growth cones extending along specific pathways, the most well-studied of which involve crossing midline structures (commissures), such as the optic chiasm and corpus callosum. Activation of these receptors determines whether an axon grows toward or away from a target cell. At least four conserved families of guidance molecules have been identified: (1) The semaphorins, which constitute a large, 20-member family of soluble, membrane-bound molecules that elicit repulsive signals through two receptor families, neuropilins and plexins; (2) the Slit family of proteins, which consists of three members in mammals and acts through Robo receptors in commissural axons to prevent them from recrossing the midline; (3) the netrin family, whose members can be repulsive or attractive for a growth cone, depending on the receptor on the axon; and (4) the ephrin family, whose members are membrane bound and interact with two families of receptors, EphA and EphB.38 In addition to regulating axonal path finding, these same guidance molecules (i.e., semaphorins, slits, netrins, and ephrins) are involved in controlling aspects of neuron migration. Upon reaching their target, some growth cones form specialized connections with dendrites to become synapses.39 Presynaptic and postsynaptic signals induce the formation and stabilization of molecules on both sides to become specialized synaptic structures.40 On the presynaptic side, for example, synaptic vesicles filled with neurotransmitter are grouped together; on the postsynaptic side, receptor molecules are grouped together into a dense domain that is sometimes located within a dendritic protrusion called a synaptic spine.41
The wiring of complex CNS systems requires a connection of multiple cell types that are located in different positions. The wiring diagram of the visual system is an example of this process that has received a massive amount of experimental attention. The retina contains primary sensory receptor neurons (rods and cones), interneurons (amacrine, bipolar, and horizontal cells), glia, and projection neurons called retinal ganglion cells. The retinal ganglion cells extend optic nerve axons that must make several choices as they proceed to their targets. As they pass the optic chiasm, axons from the temporal retina do not cross, whereas axons from the nasal retina do cross. Intrinsic signals that distinguish nasal and temporal cells (brain factor 1 and 2 transcription factors) help guide the growing axons. Upon exiting the chiasm, the optic axons grow caudally toward their two main targets: the thalamus and the superior colliculus. Branches perpendicular to the optic tracts enter the visual center of the thalamus and form synapses with the lateral geniculate nucleus (LGN). Other optic axons continue caudally to the midbrain into the superior colliculus, where they are sorted into a retinotopic map by the ephrin family receptors.
In the LGN, the optic axons form another retinotopic map. In higher mammals, the LGN is a laminar structure, with each layer connected to only one eye. During development, however, axons from both eyes have processes extending into many LGN layers. Neuronal activity related to visual experience is required for pruning back synapses and for axonal branches to segregate into layers specific for one eye or the other. The projection neurons in the LGN send axons anteriorly into the telencephalon, in which they traverse the striatum in the internal capsule and enter the cerebral cortex. The thalamocortical axons enter the cortex while neurogenesis is still actively occurring and grow into a layer called the intermediate zone that is interposed between the proliferative zones (ventricular zone and subventricular zone) and mantle zones (cortical plate). The thalamocortical fibers then innervate specific regions of neocortex. The neocortex is subdivided into functionally distinct areas, each with its own thalamic inputs. Primary visual cortex receives LGN axons. Cortical maps from other thalamic nuclei determine primary sensory cortex (e.g., auditory cortex), whereas other regions of cerebral cortex are described as associative, because their connections are primarily to other areas of cortex. In humans, some of these associative areas are both enormous and complex, including the dorsolateral prefrontal cortex, which is involved in executive function, and the ventromedial prefrontal cortex, which is involved in complex emotional/social reasoning. Both regions have late and lengthy developmental schedules, and maldevelopment of each is implicated in numerous psychiatric disorders (see Fuster42).
EXPERIENCE-EXPECTANT DEVELOPMENT
Although numerous examples of neural plasticity have been found in mammalian species, we have proposed that much of plasticity can be classified into two basic categories. Experience-expectant development involves a readiness of the brain to receive specific types of information from the environment. This readiness occurs during critical or sensitive stages in development during which there are central adaptations to information that is reliably present for all members of the species. This information includes major sensory experience, such as patterned visual information that drives the LGN axonal withdrawal described previously, and information affecting social, emotional, and cognitive development. The overproduction of neural connections is one aspect of the brain’s readiness to receive this expected information, of which a subset is selectively retained on the basis of experience.
A general process observed in many mammalian species is that a surplus of connections is produced, a large portion of which is subsequently eliminated. Evidence for overproduction and partial elimination of synapses during development has been found in many brain regions and species, including cats,43 rodents,44 monkeys,45,46 and humans.47 The overshoot in the number of synapses produced in cortical areas in many animals, including humans, has been estimated to be approximately double the number found in adults48 (see Huttenlocher49 for review). In humans, synaptic density and estimates of total synapse numbers in the visual cortex reach a peak at approximately 8 months of age, and synapse numbers decline thereafter.48 Another important finding by Huttenlocher50 is that the blooming and pruning of synapses in the frontal cortex is substantially delayed; its peak occurs during childhood. Although synapse density and absolute synapse number may differ, depending on other tissue elements, we assume for purposes of this discussion that they are equivalent. A measure that has been interpreted as reflecting synapse overproduction and loss is the volume of regions of the human cerebral cortex and other brain areas, measured by structural magnetic resonance imaging.51 Heterosynchrony (i.e., staggered developmental timing with late development of prefrontal cortex) is unique in humans among the primates.51 The clinical implications of late-developing prefrontal cortex are discussed in a later section.
The process of overproduction and selective elimination of synapses appears to be a mechanism whereby the brain is made ready to capture critical and highly reliable information from the environment. This possibility is supported by several lines of research (described in the following sections) indicating that the pruning into structured patterns of functional neural connections requires appropriate patterns of neural activity that are obtained through experience. These events occur during known critical or sensitive periods. Furthermore, the pruning appears to be driven by competitive interactions between neural connections, so that inactive neural connections are lost and connections that are most actively driven by experience are selectively maintained. “Most active” may refer to synchronous or correlated activation, such as presynaptic activity coincident with postsynaptic activity, as first proposed by Hebb,52 or some mechanism other than the mere frequency of firing. In many cases, it appears that these plastic neural systems have evolved to take advantage of information that could be “expected” for all juvenile members (i.e., it has an adaptive value for the whole species, not just individuals). In many of the experiments described in this section, investigators used interventions that disturb some aspect of the “expected” experience, leading to substantial disruptions of further development. Many patients with developmental and behavioral disorders have had similarly disturbed experiences with subsequently disrupted development.
Visual Deprivation Experiments
Studies of the effects of early visual deprivation have provided some of the strongest examples of experience inducing neural structure during development. Together, they indicate a direct link between patterns of experience-expectant visual information and patterns of neural connectivity. Experimental visual deprivation falls into two main classes. Binocular visual deprivation can be complete, depriving animals of all visual stimuli, or partial, depriving animals of patterned visual stimuli but allowing diffuse, unpatterned stimulation. This deprivation may be achieved, for example, by suturing both eyelids shut (complete deprivation) or by raising animals in complete darkness (partial deprivation). Partial deprivation reduces or distorts visual experience in some manner but allows some effect of experience on neural activity. Complete deprivation in both eyes leads to a loss in complex visuomotor learning and in the precision of neuronal response properties, but it preserves balance in eye dominance and basic perceptual skills.53 In contrast, selective deprivation in one eye during the critical period leads to a drastic reduction in its control over visual cortex neurons and behavior, whereas the nondeprived eye correspondingly gains in control. The degree of recovery from deprivation depends on the species and on the onset and duration of the deprivation period.
Binocular Deprivation
Studies of binocular deprivation have shown that appropriate visual stimulation during certain developmental stages is critical for the development of normal neural connectivity in the visual system. Dark rearing or bilateral lid closure in developing animals results in behavioral, physiological, and structural abnormalities in visual pathways.54–56 The severity and reversibility of the visual impairments are dependent on the onset and duration of the deprivation, corresponding to defined sensitive periods of a given species.57 Even short periods of early visual deprivation can result in impairments in visuomotor skills, such as visually guided placement of the forepaw in cats.58 The structural effects of dark rearing include smaller neuronal dendritic fields, reduced spine density, and reduced numbers of synapses per neuron within the visual cortex.43,59–61 In kittens, for example, developmental binocular deprivation resulted in a 40% reduction in the number of adult visual cortex synapses.43
Selective Deprivation
Experiments in selective deprivation have indicated the importance of specific types of visual experience to normal brain development. For example, kittens reared in a strobe-illuminated environment have plentiful visual pattern experience but are selectively deprived of the normal experience of movement (i.e., movement in the visual field would appear jerky or disconnected). Specific impairments in motion perception have been found in such kittens.62 These animals had visual cortical neurons that were insensitive to visual motion,63 and they exhibited impairment on visuomotor behavioral tasks that involve motion.64
Other researchers limited visual experience to specific visual patterns, or contours. Hirsch and Spinelli65 raised kittens in chambers with one eye exposed to only horizontal stripes and the other eye to only vertical stripes. Physiological recordings of visual cortical neurons from these kittens revealed that neurons were most responsive to stimuli oriented in the direction of the stripes they had experienced. These neurons also occupy twice as much of the visual cortex as neurons sensitive to stripes in nonexposed directions.66 Behaviorally, stripe-reared animals performed best on tests involving stimuli in the orientation to which they were exposed during development.67,68 Unlike dark rearing or bilateral lid closure, stripe rearing does not appear to result in an overall diminishment of neuronal size, but it does alter the orientation of the neuronal dendritic arbors.69,70 Thus, neural function appears to be determined by the pattern, in addition to the overall number, of neural connections. A related, albeit debatable clinical finding in humans who have uncorrected astigmatism in a particular orientation in one eye is reduced acuity in that axis.71
Monocular Deprivation
A great deal of information about experience-expectant processes has been learned from one particular deprivation model. In species with stereoscopic vision, including cats and monkeys, binocular regions of the cortex receive information from each eye via projections from the LGN in adjacent stripes or columns within cortical layer IV, termed ocular dominance columns. With normal experience early in development, the cortical input associated with each eye initially projects in overlapping terminal fields within layer IV. During development in normal animals, these axonal terminal fields are selectively pruned, which results in sharply defined borders between ocular dominance columns in adult animals. The neurons of this layer send convergent input to other layers, made up in large part by binocularly driven neurons.72
Studies of monocular deprivation in stereoscopic animals have shown that the formation of the ocular dominance columns is dependent on competitive interactions between the visual input from each eye.73 In monocularly deprived monkeys, the axons projecting from the deprived eye regress, whereas the axons from the experienced eye do not. This pruning back results in the thinning of the columns corresponding to the deprived eye, whereas the columns of the nondeprived eye are enlarged in relation to those of normal animals.72,74 Thus, the axonal terminals from the dominant eye appear to be selectively maintained at the expense of the inactive input of the deprived eye, in which the excess synapses are eliminated. Physiologically, the number and responsiveness of cells activated by the deprived eye are severely decreased.55 Functionally, monocular deprivation for an extended period during development results in near blindness to visual input in the deprived eye. In contrast, binocular deprivation results principally in a loss of visual acuity. Physiologically, it reduces but does not abolish the response of neurons to visual stimuli.55 It also does not prevent the formation of ocular dominance columns, although the segregation of columns is well below normal.72,75,76 Thus, in binocular deprivation, cortical input from the eyes may be partially maintained in the absence of competing information.
The physiological and anatomical effects of monocular deprivation occur fairly rapidly. Antonini and Stryker74 found that the shrinkage of geniculocortical arbors corresponding to the deprived eye was profound in cats with only 6 to 7 days of monocular deprivation, similar to that found after 33 days of deprivation. Like binocular deprivation, the recovery from the deprivation is sensitive to the time of onset and duration of the deprivation. Monocular deprivation corresponding to the sensitive period of a given species results in enduring impairments and physiological nonresponsiveness,55 whereas even very extensive deprivation in adult animals has little effect.77 In humans, early monocular deprivation resulting from congenital cataracts can have severe effects on acuity, even after treatment, whereas adults who develop cataracts in one eye show little post-treatment impairment.78 The sensitive period for the effects of monocular deprivation can be affected by prior experience. For example, the maximum sensitivity to monocular deprivation in kittens is normally during the fourth and fifth weeks after birth.79,80 Cynader and Mitchell81 found that kittens dark-reared from birth to several months of age maintain a physiological sensitivity to monocular deprivation at ages that normal kittens are insensitive. Dark-reared animals do not, however, simply show normal visual development at this later age. With binocular deprivation early in life, the ocular dominance columns of layer IV do not segregate in a fully normal pattern and do not maintain a structural sensitivity to monocular deprivation effects.75
The implication of these studies is that the sensitive period for experience effects is self-limiting; that is, as supernumerary synapses are eliminated and/or as additional synapses cease to be generated, the capacity for responding, or the experience-sensitive phase, comes to an end. Alternative models might invoke changes in the synapses that survive, leaving them immutable to further pruning or to forces acting at a distance, such as the development of local GABA-based inhibitory systems82,83 or the influence of modulatory axonal activity from other parts of the brain. There are a variety of such proposals, many with supporting data (e.g., α-amino-3-hydroxy-5-methyl-4-isoxazolepropionic acid [AMPA] and N-methyl-d-aspartate [NMDA] glutamate receptor distribution and subunit composition84,85
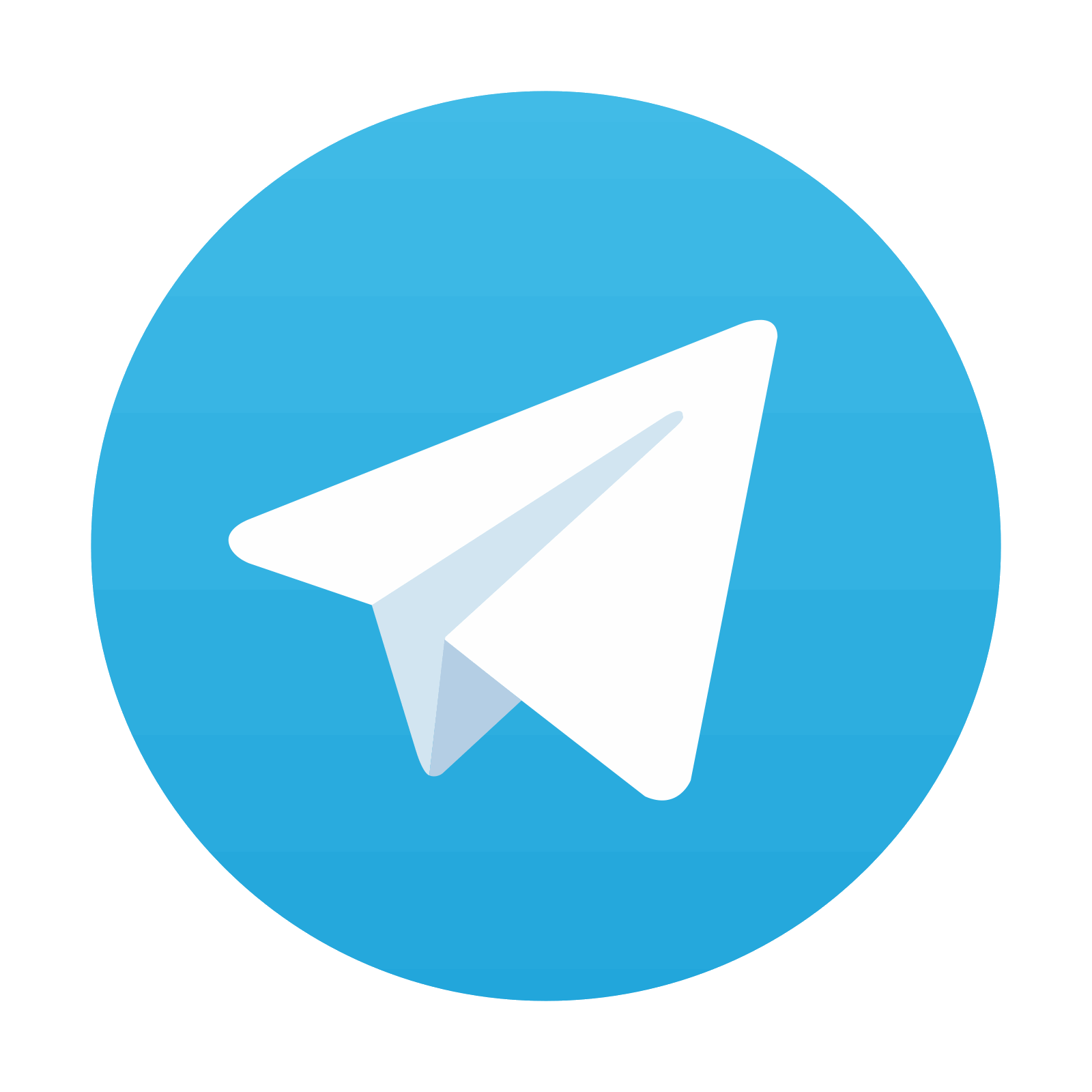
Stay updated, free articles. Join our Telegram channel
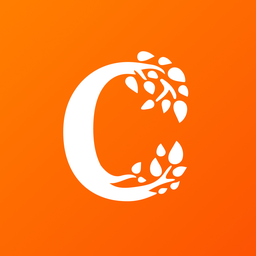
Full access? Get Clinical Tree
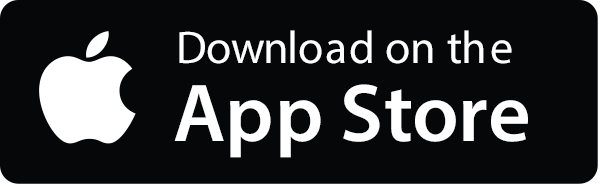
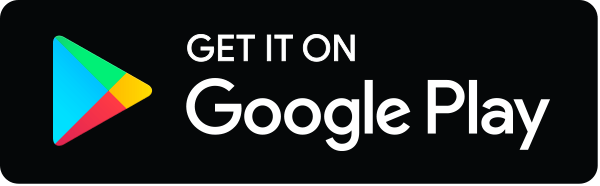