The spectrum of visible light; numbers on the left represent wavelength in nanometers. Ultraviolet light has wavelengths between approximately 310 nm and 100 nm, with photon energies from 4 eV to 12.4 eV. Wavelengths in the 400–300 nm range are known as “near ultraviolet” (visible to birds, insects, and fish), and have photon energies of 3.10–4.13 eV. At the other end of the spectrum, infrared (IR) light wavelengths extend from the red edge of the visible spectrum at 700 nm to 1 mm, with photon energies 1.24 mEV–1.7 eV.
Most of the thermal radiation emitted by objects near room temperature is infrared radiant energy.
Type | Source | Comments |
---|---|---|
Sunlight | Windows | Includes 400 t0 500 nm |
Fluorescent | Indoor lighting | 440 to 500 nm via mercury arc |
Compact fluorescent | Indoor lighting hoods | 400 to 700 nm |
Incandescent | Indoor lighting | Some light in 400 to 500 nm |
Halogen | Indoor lightingMicroscopes | Depends on bulb; can be coated to filter out wavelengths |
LED | MicroscopesIndoor lighting | Depends on LED type |
Mercury arc | Microscopes | 300 to 600 nm range |
Is it practical or possible to reduce the wavelengths in the critical spectra – 400 to 500 nm, without compromising patient and technician safety? Under variable, but working lighting conditions, Ottosen and colleagues demonstrated that plastic polystyrene dishes did reduce or absorb small, but not significant amounts of energies in the 400 to 500 nm spectra. Short wavelength filters applied to halogen bulb microscopes and room bulbs (background fluorescent and natural lighting) significantly reduced the light energies in this critical spectral range. Duration and intensity of light exposure were also considered important, and were modeled according to the preference of each technician. The authors concluded that reducing ambient light intensity (while maintaining light intensity comfortable for the technicians), reducing duration of light exposure to gametes and embryos, and specifically targeting microscope lighting by adding red or amber filters would provide the greatest benefit.
Using a green bypass filter (such as the Schott VG-9) could provide a simple strategy to reduce embryo light exposure from a microscope, until the effect of light on human embryos is better understood. A green bypass filter can protect embryos from deleterious effects of both blue and near infra-red wavelengths. A green filter is more effective than red, as the human eye is more sensitive to green than to red (Parr, 2001).
From a practical standpoint, lighting in the laboratory should be sufficiently bright for personnel safety, at the same time ensuring the safety of the gametes and embryos handled in the laboratory. Blue wavelength filters create a visible spectrum perceived as amber to yellow light; in this environment, color labels can be washed out or altered, pH monitoring via phenol red in culture medium can be compromised, and the filters reduce the intensity of available lighting, making it necessary to employ additional incandescent lamps in some laboratories.
Reactive oxygen species
Potentially negative effects of light on ova and embryos can be reduced via two strategies: (1) reduce exposure to light via filters on microscopes and light fixtures to remove the blue spectrum (mentioned above) and (2) mitigate damage from light-induced ROS by adding antioxidants to culture media.
ROS are generated as an intrinsic consequence of cell metabolism, e.g. mitochondrial function, where the potentially damaging nature of these molecules is balanced by the inherent antioxidant capacity of the cells. When this balance is interrupted, an overabundance of more potent radicals (hydroxyl and H2O2 compounds) can damage cells, specifically targeting lipids and DNA. Gametes and embryos in vitro are exposed, transiently or continuously to supra-physiological oxygen, temperature and pH excursions; exposure to various light energies can be responsible for adding to the total oxidative cell load in vitro. Male and female reproductive cells are sensitive to increased ROS exposure in vivo and in vitro, and in some cases, reproductive potential may be impaired (Agarwal & Allamaneni, 2004; Agarwal et al., 2006).
The specific targets for damage-inducing ROS compounds in vitro has been debated. Mammalian sperm plasma membrane lipids can be damaged following light-induced increased ROS production. Oxygen radicals were produced in significantly greater quantities following exposure of sperm membranes to blue spectra (400–505 nm) compared to red spectra (600–800 nm); the authors speculate that the mechanism involves membrane lipids directly, and not via an external catalyzed process (Lavi et al., 2012). Ram sperm were exposed to white light (400–800 nm), red light (660 nm), blue light (360 nm) and UV light (294 nm) in vitro. Processed, light-exposed sperm were then used for in vitro fertilization. Motility of ram sperm was compromised by exposure to white, blue, and UV spectra, and embryo viability after IVF was highest for sperm exposed to control (darkness) and red spectra. Additionally, lower ROS concentrations were generated by exposure to the longer (red) spectral wavelengths (Zan-Bar et al., 2005).
As previously mentioned, light has been linked to increased production of ROS and to DNA damage. Human embryo fragmentation (Yang et al., 1998) has been linked to apoptotic processes, which may be affected in vitro by ROS production; furthermore, ROS production increases as oxygen tension increases. The detrimental effect of increased ROS may be manifested at the mitochondrial level; the antioxidative capacity of the culture medium and the embryo itself may counter some of these negative ROS effects (Catt & Henman, 2000; Guerin et al., 2001). It is thus possible to conclude that ROS production may be additive, e.g. combining non-physiological oxygen tension with ambient light exposure might together jeopardize the development of human embryos in vitro (Noda et al., 1994).
Oocytes and embryos have some capacity for intrinsic DNA repair, but the sperm cell does not (Ménézo et al., 2007; Ménézo et al., 2010). Protecting cells in vitro – sperm, oocytes, and embryos – involves minimizing environmental stressors; the culture environment contributes to oxidative damage, and inclusion of antioxidants in the medium may provide additional protection (Bavister, 2000; Guerin et al., 2001; Martín-Romero et al., 2008; Moshkdanian et al., 2011; Orsi & Leese, 2001). Altering the ability of the oocyte and embryo to repair DNA that has been damaged by exposure to increased ROS can lead to altered transcription of repair mechanisms, and potentially reduced fertility (El-Mouatassim et al., 2007).
Therefore, culture system design should focus on minimizing opportunities for additional ROS production. A systematic approach to designing an embryo culture system includes choosing from the many commercial culture media available (although some clinics still manufacture their own culture media in-house); this is one of the more critical aspects of human clinical IVF. Culture media for human embryos have evolved significantly over the years, and yet unsubstantiated and often misinterpreted concepts regarding culture medium components and phototoxicity have been perpetuated over the years, stemming from several early tumor cell culture studies. For example, Spierenburg’s team found that cell culture media that were not formulated for use with mammalian embryos in vitro containing HEPES and riboflavin compounds (B2 vitamins), but not pyruvate, were vulnerable to fluorescent light-induced peroxidation in the presence of oxygen (Spierenburg et al., 1984). The protective role of pyruvate against downstream light-induced peroxidation toxicity in culture media may be via its action as an oxidative scavenger, thus maintaining intracellular glutathione concentrations (Babich et al., 2009).
Current culture media for human and other mammalian embryos typically contain pyruvate; some contain some or all essential and non-essential amino acids, but they do not usually contain riboflavin compounds, which are responsible for light-induced phototoxicity (Edwards & Silva, 2001). However, significant ROS generation may still occur simply by warming the medium to incubator temperatures (Martín-Romero et al., 2008).
Quality of the oil used as a culture medium overlay is an additional important and often overlooked component of the culture system. A commercial oil product may arrive sterile from the manufacturer with a valid certificate of analysis, but improper transportation temperature, exposure to sunlight and/or visible light or improper storage may result in adulteration of the oil (see Chapter 3). Peroxides can form in a cascade reaction as a result of exposure to light and/or heat, with transfer of water soluble contaminants to the culture drops (Otsuki et al., 2009, 2007; Provo & Herr, 1998).
It is clear that ROS generation in vitro is unavoidable; addition of exogenous antioxidants (e.g. ascorbic acid), and supporting gamete and embryonic health to drive intrinsic antioxidant capabilities may counter the more damaging effects of ROS exposure. Technologies already exist to limit external stressors, for example, supraphysiological oxygen concentrations can be avoided by using a specific incubator design to reduce oxygen concentrations, and pre-mixed low oxygen concentration gases are available for use with mini-incubators and enclosed containers. Blue wavelength light energies can be avoided altogether by selecting lighting sources and/or use of light filtration devices; exposure of gametes and embryos to any light energies can be minimized by limiting the time that these cells are maintained outside of the incubator. Careful selection and storage of embryo culture media and oil for overlays can contribute to reducing ROS exposure. Nonetheless, even if damage from ROS is avoided via some of the methods above, damage from light that is not mediated by ROS is still possible.
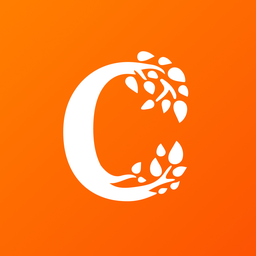
Full access? Get Clinical Tree
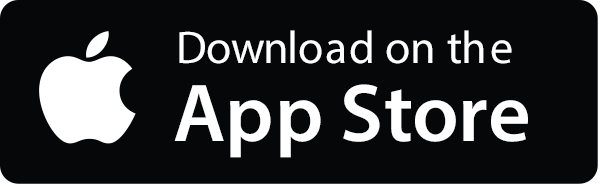
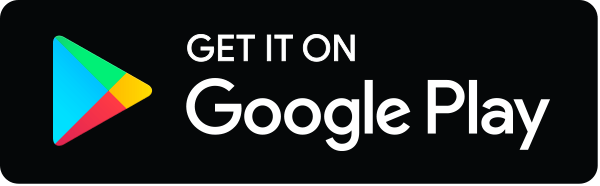