Drugs commonly used in intensive care [12], such as morphine, midazolam, and vecuronium, all produce active metabolites that are dependent on adequate renal elimination. Morphine is converted in the liver to morphine-6-glucuronide, which is very active at opioid receptors, and morphine-3-glucuronide, which is active at NMDA receptors and is excitant and may be pro-convulsant [13, 14]. Midazolam is metabolized to alpha1-hydroxymidazolam, which, although less potent than the parent compound, has sedative activity and both compounds may accumulate [15]. Vecuronium has several active metabolites 3- and 17- hydroxyl- and 3,17- hydroxyl-vecuronium. All such compounds require renal elimination and thus often accumulate in the ICU patient. Consequently, there is a real danger of residual paralysis after cessation of muscle relaxants. Although this can be assessed using train-of-four nerve stimulation, caution must also be exercised in attempting to reverse this with sugammadex. Although sugammadex will clear the “accessible” ECF, it will not bind with drug that has crossed into other tissues. This enlarged volume of distribution is thought to be a function of disturbed capillary function in critical illness and length of time receiving the drug [16].
Another complicating factor is the post-conceptual age (or “postmenstrual” age [PMA]) of infants. The size of the child is only one consideration where other variables are important such as PMA and neurological maturation affecting pharmacodynamics and the pharmacokinetics affected by continuously developing organ maturation, although these factors are far from being fully understood [17, 18]. In infants with no renal compromise, glomerular filtration rates (GFR) have been studied. They appear to be at about 30 % of adult values at term and rise to approximately 90 % by 1 year of age, but the GFR of preterm infants may only be approximately 10 % [19].
A workaround for this complex situation may be to electively cease sedative infusions at a set time each day, which allows the patient to clear drugs, reduce accumulation in a variety of pharmacokinetic compartments, and emerge from sedation. This permits a practical recalibration of the level of sedation with the drugs being administered and prevents relentless loading of the patients’ pharmacokinetic compartments. The clinical application of this in an adult ICU population was demonstrated by Kress where such interruptions were associated with decreased durations of ventilation and length of stay in the ICU [20]. More recently this has been reproduced in a pediatric population and, importantly, without any increase in adverse events, such as respiratory complications or accidental extubations [21].
Recent studies also suggest that the benefits of daily interruption of sedation can also be seen with nurse-led sedation protocols. It is possible that a combination of both strategies may lead to most benefit [22]. Generally, the greater the analgesia and sedation used, especially if this is combined with a degree of delirium, then the greater the length of time on the ventilator and in the ICU. This can be ameliorated if structure is given to sedation and analgesia administration [23]. Even where protocolization of sedation and analgesia resulted in greater doses of drugs being administered to neonates, it did not lead to increased durations of ventilation, length of ICU stay, or adverse outcomes [24]. This may be because there was greater structure for timeliness of increasing doses but also for decreasing them in conjunction with non-pharmacological measures.
Another strategy studied is the effect of automation on effective sedation ICU patients. Le Guen found that, when targeting a range of 40–60 bispectral index (BIS) using remifentanil and propofol, an automated system using a closed-loop controller linked directly to the BIS outperformed the staff-controlled system attempting to track the BIS manually [25]. This may indicate another avenue worthy of future study in the pediatric population.
Specific Aspects
Opioids
In the general population as a whole, there is an increasing appreciation that widespread complex genetic variation affects opioid absorption, distribution, metabolism, excretion, and toxicity [26]. This means that assumptions about drug effects and their elimination should be treated with caution.
The maturation of renal clearance of morphine in infants born preterm does not normalize at postmenstrual age of 40 weeks (i.e., term) but occupies a separate trajectory from those infants born at term [18]; thus, the administration of morphine to infants born preterm should reflect this difference in pharmacokinetics.
It has long been recognized that opioids induce tolerance and the need to increase doses to produce the same desired effect [27]. This is either “tolerance” where the μ-opioid receptor complex becomes desensitized, or “tachyphylaxis” where compensatory physiology—for example, activation of antagonist signaling systems such as the N-methyl-d-aspartate (NMDA) pathway—then runs unopposed when the opioid is removed. Whatever the underlying mechanism is, the acute removal of the opioid will thereby lead to withdrawal symptoms [28].
This effect of acute tolerance may well be more pronounced when the opioid has greater affinity for the opioid receptor, and this has been documented with the use of remifentanil [29, 30]. Theoretically, inhibition of the NMDA pathway with an agent such as ketamine may be expected to decrease the induced “compensatory” antagonism caused by that pathway against opioid effects at μ-receptors, but data from a study in children undergoing scoliosis surgery failed to demonstrate any benefit from co-administration of low-dose ketamine [31].
Benzodiazepines
Overall there is increasing evidence that benzodiazepine-based sedation is associated with longer length of stay and duration of ventilation, compared to non-benzodiazepine sedation regimens [32] and the introduction of propofol to adult ICU practice has been associated with shorter times on ventilation and decreased length of stay [33]. However, the use of propofol in pediatric practice remains low due to the concerns about outcomes associated with its use [34–38].
Midazolam remains one of the most commonly used benzodiazepines in ICU practice in both adults [39] and children [12], but there is a growing recognition that it is associated with significant morbidity. It is possible to measure plasma levels of midazolam and its metabolite alpha-1-hydroxymidazolam, but the concentration of these substances at which satisfactory clinical sedation is achieved varies sufficiently to preclude this form of monitoring from being practically useful [15].
Ketamine
Ketamine preparations may exist as one of two enantiomers, S(+)ketamine and R(−)ketamine. The S+ version is more potent and may have less side effects, and may be available singly, but the most prevalent preparation is still the mixed racemic R−/S+ form [43].
Ketamine, like other sedative agents, has been implicated in neurone apoptosis when used in infants. One study confirmed this, but by using large doses of ketamine in immature rates (20 mg kg−1) [44]. Interestingly, this effect could be blocked either by the administration of vitamin D3 or by “preconditioning” with ketamine at 5 mg kg−1, a dose closer to that used in clinical practice.
However, there is also a possibility that ketamine may be neuroprotective in situations where neurological insults may be expected, as in cardiopulmonary bypass or frank hypoxic–ischemic damage, where resulting glutamate toxicity that would be mediated through NMDA receptors may be blocked by ketamine [45]. Antagonism of NMDA receptors also decreases the amounts of opioid necessary to attain satisfactory analgesia postoperatively [46] and decreases the rebound hyperalgesia ascribed to opioid tolerance induced by fentanyl and morphine [47].
There have been concerns that the use of ketamine in encephalopathies or traumatic brain injury might increase intracranial blood pressure (ICP) and thus compromise the injured brain [48]. However, these fears may not be well founded [49]. In 1997, Albanese and colleagues showed that incremental doses of ketamine, given to patients with intracranial monitoring, actually decreased the ICP [50]. Subsequent studies have similarly failed to show any detriment attributable to ketamine even if direct benefit has not been demonstrated [51].
The use of ketamine in asthma has also been evaluated. There is anecdotal evidence that ketamine may avert the need for intubation and ventilation at higher doses [52]. In a recent Cochrane review, only one study in children was eligible for inclusion, highlighting the paucity of good data in this area [53]. This study was conducted in unintubated children with relatively low doses of ketamine; a bolus of 0.2 mg kg−1 followed by an infusion at 0.5 mg kg−1 h−1 and showed no benefit in terms of hospital admission rate or need for mechanical ventilation [54].
It has been shown that ketamine does not raise pulmonary vascular resistance in children with cardiovascular disease and in those with preexisting pulmonary hypertension [55].
After an early case report of possible effectiveness of ketamine in resistant status epilepticus using quite low doses (a bolus of 2 μg kg−1 followed by 7.5 μg kg−1 h−1) [56], a more recent study of children and adults with resistant status epilepticus has identified a possible role for ketamine in treating this disorder. The rationale for its potential effects is based on the fact that prolonged seizures are accompanied by a decline in effects from gamma-aminobutyric acid agonists (benzodiazepines) but not to NMDA antagonists [57]. There appeared to be some benefit, but only with doses superior to 0.9 mg kg−1 h−1.
Inhalational Sedation
Isoflurane has been trialed over the last 20 years as an ICU sedative agent, particularly with interest in its possible beneficial effects in the management of asthma [58], but has proved itself to be free from neither logistic difficulties in delivery by ventilators adapted to deliver the agent with necessary scavenging [59, 60], nor in freedom from withdrawal phenomena [61].
The “Anaconda®” system (Sedana, Uppsala, Sweden) has been utilized to administer either isoflurane or sevoflurane and conserve the agents by “reflective” rebreathing. Unfortunately this adds 100 ml to the dead space, precluding its use in smaller children unless the device is moved to lie wholly within the inspiratory limb of the ventilator circuit [60, 62, 63]. However, this negates the rebreathing element of the device and raises consumption. End-tidal monitoring of the agent must be monitored and this is titrated against flow rates of liquid agent infused directly into the device using nomograms available as a guide. For isoflurane, end-tidal concentrations of isoflurane varying from 0.6 % to 1.2 % have been utilized [60, 62].
Both sevoflurane and isoflurane are metabolized within the body and cause fluoride ions to be excreted in the urine with the potential for renal tubular toxicity, particularly with more prolonged administration in the ICU. However, there has been little evidence of renal toxicity in studies of isoflurane in the ICU [64].
Xenon is proving to be an interesting element in regard to its use as an inhalational anesthetic and sedative agent. However, its anesthetic properties have been known since 1951 [65], including a very low blood-gas partition coefficient (leading to faster induction of effect and emergence), and with both hypnotic and analgesic effects. There are interesting and debatable aspects additional to these obvious advantages, when compared to the hydrofluorocarbon anesthetics; potential for greater cardiovascular stability [65], neuroprotection, and lack of greenhouse and ozone effects to be balanced against the increased costs in its production and utilization [66].
When isoflurane anesthesia was compared to xenon anesthesia in a multicenter trial, it was noted that xenon was safe, effective, and provided quicker recovery [67].
In a direct comparison to an intravenous propofol-alfentanil regimen, inhaled xenon at a mean inspired fraction of 28 % (range 9–62 %) provided satisfactory sedation with more cardiovascular stability and faster recovery times [68]. When xenon was compared to total intravenous anesthesia for adult vascular surgery, xenon was associated with dampening of autonomic responses [69].
Xenon has been shown to have neuroprotective effects by limiting the measurable effects of hypoxic–ischemic encephalopathy [70] and this has been borne out by histopathologic findings [71]. It is thought that xenon may produce its neuroprotective effect by competitive binding at the glycine site of the NMDA receptor [72].
In a prospective study on adult survivors of out-of-hospital cardiac arrests, it was found that xenon administered in hypothermic management reduced both markers of cardiac damage and cardiovascular instability [73].
While all the properties alluded to above may make xenon appear an attractive future anesthetic for cardiopulmonary bypass cases, it is equally capable of dissolving into any nitrogen bubbles in the circuit, in much the same way that has long been recognized for nitrous oxide. Thus, notwithstanding its demonstrated neuroprotective effects, this event would exacerbate “air” embolism [74] and, at least experimentally, this has been borne out with histopathology study [75].
It may have been hoped that the use of inhalational agents may have circumvented mechanisms causing withdrawal that was so evident from the intravenous agents. However, it became clear, that whatever the agent, compensatory mechanisms arise in the body to develop both tolerance and a degree of dependence, including isoflurane [61].
Alpha-2 Receptor Agonists
These agents work at several disparate sites where alpha-2 receptors exist [76]: presynaptically at sympathetic nerve endings (sympatholysis) [77], within the substantia gelatinosa [78] and affecting substance P release [79] (analgesia) and more centrally on the locus coeruleus (sedation and analgesia) [80] and potentially the nucleus ambiguus and dorsal motor nucleus of the vagus nerve (parasympathetic stimulation) [81]. The side effects that were anticipated as potential problems consequent to use in intensive care were primarily hypotension and bradycardia.
There is increasing information about the use of alpha-2 receptor agonists as sedatives in the ICU. The ones mostly studied in the ICU and PICU have been clonidine and dexmedetomidine, with the latter attracting the greater attention. It has eight times the affinity of clonidine for the alpha-2 receptor [82] while having a half-life of 2–3 h compared to 12–24 h, respectively. The metabolic breakdown product of dexmedetomidine, “the H-3 metabolite,” is thought to have only 0.5 % of the pharmacodynamic activity of the parent compound, which adds to the safety profile [83].
However, the effects of distribution on context-sensitive half-lives for both drugs have to be borne in mind, as indeed for many other sedatives in common use. When dexmedetomidine levels were measured in a cohort of critically ill patients, increasing the infusion rate to 2.5 μg kg−1 h−1 failed to show any accumulation and indeed circulating levels showed linearity with respect to the infusion rate [83]. However, the investigators point out that patients with known hepatic dysfunction were not included in this cohort so there remains a caveat in this regard.
When studied as an infused sedative of greater than 48 h duration, with loading of 3–6 μg kg−1, followed by a modest rate of 0.1–0.25 μg kg−1 h−1, its clearance was reduced in patients with decreased cardiac output and increasing age, and its volume of distribution was increased with hypoalbuminemia. Modeling suggested that context-sensitive half-times would be increased under these circumstances [84].
However, licensing processes, which vary internationally, have affected the distribution of use of dexmedetomidine in children. When the pharmacokinetics of clonidine in children were studied, it was found that clearance is dependent on renal function, with about 50 % being renally excreted unchanged and 50 % undergoing hepatic transformation. The clearance will therefore be dictated by renal function and, for small infants, on renal maturity [85]. In addition it was also found that the context-sensitive half-life may double with prolonged infusions, this being a function of its high lipid solubility and thus sequestration in peripheral tissues [85].
In an early study of the dose–response for clonidine in the PICU, it was found that doses of up to 2 μg kg−1 h−1 of clonidine when combined with midazolam at 50 μg kg−1 h−1 produced acceptable sedation and analgesia without adverse effects on cardiovascular performance, even after cardiac surgery [86]. Subsequently, also in a heterogeneous population of ventilated PICU patients, enterally administered clonidine 3–5 μg kg−1 8 hourly was found to give adequate sedation, but this too was given with a background of both a benzodiazepine (in this study lorazepam) and morphine intravenously. However, there seemed to be increased sparing of both agents with time when combined with clonidine in this manner [87]. A multicenter, randomized, blinded trial comparing midazolam and clonidine has been carried out in the UK and results are awaited [88].
One emerging area is the possible benefits of alpha-2 agonist sedation in reducing delirium and other cognitive disorders associated with intensive care. When compared to lorazepam in adults, dexmedetomidine was shown to be associated with significantly less delirium and deep unconsciousness [89]. Additionally, the dexmedetomidine group appeared to have better preserved cognition in post-ICU neuropsychological testing.
When dexmedetomidine was compared to midazolam, there were similar findings; incidence of delirium and times to extubation were more favorable in the dexmedetomidine group. While bradycardia was more frequent in the dexmedetomidine group, the extent to which this needed treating was not significant statistically and the incidence of tachycardia and hypertension needing treatment was lower with dexmedetomidine [90].
When compared to propofol, dexmedetomidine is better at preserving cognition and “cooperative sedation” and thus may prevent the emergence of delirium [91].
When the dosing was increased to over 0.7 μg kg−1 h−1 in trauma patients, then hypotension was more frequent and hypertension was occasionally seen with loading [92]. This is thought to be due to the action of the different responses to stimulation of the two subtypes of alpha-2 receptors (alpha-2a and alpha-2b) producing hypotension and hypertension, respectively, and where alpha-2b-mediated activity is seen at the higher doses [93]. A dosing protocol in adults, titrating the infusion rate versus the Ramsay Sedation Score only every 30 min and not exceeding 0.7 μg kg−1 h−1 reduced the incidence of hypotension fourfold [93].
While it has been known for some time that clonidine does slow gastrointestinal transit [94], dexmedetomidine at 0.2 μg kg−1 h−1 appeared to be free from this side effect [95]. However, when given at the higher range, 0.7 μg kg−1 h−1, there was quite a marked effect on slowing transit compared to morphine [96]. Thus, one potential advantage of this agent compared to opioids may be negated.
A large international multicenter study of dexmedetomidine use in adults has been carried out: one arm comparing it with midazolam, the other arm with propofol [97]. It was observed that dexmedetomidine provided equivalent quality of sedation compared to the other two agents and shorter duration of mechanical ventilation in the comparison with midazolam. Durations of ventilation were not significantly different in the comparison with propofol, but in both groups the time to extubation was shorter with dexmedetomidine. The ability of patients to interact with ICU staff appeared to be better with the use of dexmedetomidine than with either of the other two agents. Hypotension incidence was higher with dexmedetomidine compared with midazolam but not with propofol, the dexmedetomidine dose range in this study being permitted up to 1.4 μg kg−1 h−1.
In a dose finding study of dexmedetomidine in ventilated PICU patients, Tobias found that a dose range of 0.25–0.5 μg kg−1 h−1 seemed to provide satisfactory conditions compared to midazolam and with the higher dose of 0.5 μg kg−1 h−1 perhaps providing more optimal conditions [98]. Doses up to 0.7 μg kg−1 h−1 were used in a subsequent observational study with little effect on blood pressure and heart rate, even when 76 % of the population studied had undergone cardiac surgery [99]. This contrasts with the findings of Hosokawa in an uncontrolled, sequential observational study in children after cardiac surgery; a mixed sedation regimen of chlorpromazine, fentanyl, and midazolam was compared with a subsequent sedative regimen based on dexmedetomidine but other agents such as midazolam, chlorpromazine, and fentanyl were added in as deemed necessary. The dexmedetomidine rate never exceeded 0.6 μg kg−1 h−1. There were statistically significantly more episodes of hypotension but also faster times to extubation and less need for noninvasive respiratory support [100].
More recently an open-label, dose escalation pharmacokinetic and pharmacodynamic study in children after open heart surgery has been reported [101]. Three loading doses over ten minutes of either 0.35, 0.7, or 1 μg kg−1 were followed by respective continuous infusions of either 0.25, 0.5, or 0.75 μg kg−1 h−1 for 24 h. The frequency with which these different cohorts received supplemental sedation or analgesia with midazolam, morphine, fentanyl, or acetaminophen did not differ between the groups with any statistical significance. The level of sedation was monitored by the University of Michigan Sedation Scale [102] and this appeared to have moderate correlation with plasma levels of dexmedetomidine. The three cohorts showed no significant differences in cardiovascular or respiratory effects except a dose-dependent reduction in heart rate, although this was not felt to represent any clinical problem. Additionally, tracheal extubation was achieved within the 24-h infusion period amongst the three groups without any significant difference. When the pharmacokinetics of these dose ranges in children after open heart surgery were examined in detail, there did not appear to be any accumulation [103].
It may be that due to its effective partial sympatholysis, perhaps mediated through parasympathetic stimulation effects, dexmedetomidine may reduce the propensity to tachydysrhythmias. There are a few reports of possible beneficial effects in children in dealing with dysrhythmias such as junctional ectopic, supraventricular, and ventricular tachycardias [81].
It is not yet clear whether dexmedetomidine is associated with withdrawal symptoms that are seen with all other sedative and analgesic agents after prolonged administration. Darnell reported a sequence of events in an infant that would seem to indicate that withdrawal to dexmedetomidine can occur [104]. When dexmedetomidine was stopped in a cohort of children after at least 3 days of receiving cardiac intensive care, tachycardia, transient hypertension, and agitation were observed [105]. Earlier reports of the use of dexmedetomidine in children and adults suggested that it may not be associated with withdrawal [106–108]. There is some question therefore as to whether dexmedetomidine can be associated with withdrawal with these reports divided at the moment [109].
Dexmedetomidine has been reported as a “rescue” treatment for sedation withdrawal symptoms in children and it can be given subcutaneously, appropriately diluted, allowing removal of intravenous devices when they would not be otherwise indicated [110].
Propofol
This drug has many good attributes for a drug for sedation in intensive care. It has a good pharmacokinetic profile with minimal elevation of context-sensitive half-life with prolonged infusions. It is associated with fairly rapid reversal of sedation after cessation of the infusion. It reduces cerebral metabolism, reduces intracranial pressure, and—provided the blood pressure is supported, as is usual in ICU—has no deleterious effects on cerebral perfusion pressure [111], and has anti-inflammatory, antioxidant, and anticonvulsant properties [112].
It was not associated with any major problems until a small series of fatal myocardial failure in children was reported. This described metabolic acidosis and fatal myocardial failure in five children across three intensive care units in the UK [36]. The clinical picture in these children was remarkably similar, the salient features being an increasing metabolic acidosis, bradyarrhythmia, and progressive myocardial failure. The average infusion rate of propofol in these children was between 7.4 and 10 mg kg−1 h−1.
At the same time, there were concerns that propofol administered as a sole agent, and thus at higher doses (6–18 mg kg−1 h−1), could result in neurologic signs following cessation of the infusions. These consisted of abnormal myoclonic and choreiform activity although there were no long-term sequelae [37]. By 1998, with the emergence of additional similar case reports, the concept of a “propofol infusion syndrome” (PRIS) was taking root [113]. Nonetheless, there was another school of thought that was skeptical regarding the existence of PRIS and claiming that at the moderate doses many employed, backed up by pharmacokinetic data, there had been no occurrence of this syndrome [114, 115].
Subsequently, studies were conducted to explore whether propofol could be given safely under controlled conditions. It was suggested that infusion rates of ≤4 mg kg−1 h−1 for less than 48 h—while assiduously monitoring acid–base, lactate, and triglyceride levels—may be safe but acknowledging that further research was needed [35]; this was the position adopted by many in pediatric intensive care [34] and backed up by prospective studies [35, 116].
Clues to the etiology started to come from metabolic studies of children affected by what was termed PRIS. Wolf and colleagues studied a 2-year-old child who sustained head trauma, was given propofol up to 5.4 mg kg−1 h−1 and then developed renal failure and nodal bradycardia. After stopping the propofol and commencing transvenous pacing, the metabolic acidosis continued to worsen. Before starting hemofiltration, blood analysis showed raised malonylcarnitine, C5-acylcarnitine, creatine kinase, troponin-T, and myoglobinemia. The acidosis and cardiac function resolved after hemofiltration.
At a 9-month follow-up, all markers of fatty acid metabolism were normal. It was postulated that propofol had interfered with entry of long-chain acylcarnitine esters into mitochondria and caused failure of the mitochondrial respiratory chain at “complex II” and that this may have been exacerbated by low carbohydrate intake (39.8 kcal kg−1 day−1) [38]. This was echoed by a subsequent case in Canada of a 5-month-old child sedated after a cleft lip repair. The carbohydrate intake had been restricted to 1.53–2.7 mg kg−1 min−1 and the propofol infusion rate had been increased sequentially to 15 mg kg−1 h−1 over 2 days. The child then developed wide complex tachydysrhythmias, metabolic acidosis, renal failure, hyperkalemia, hepatic dysfunction, and hypertriglyceridemia. Within 2 h of charcoal hemoperfusion the cardiac abnormalities showed marked improvement. Blood analysis prior to hemoperfusion also showed markedly abnormal acylcarnitine chemistry [117]. Further work also reinforced the notion that doses of propofol beyond 4 mg kg−1 h−1 seemed to be associated with the appearance of abnormal acylcarnitine biochemistry [118].
More recently, fatal PRIS occurred in an adult who had an underlying deficiency in skeletal muscle oxidative pathway, thus reinforcing the theory that the etiology of PRIS is likely to be due to abnormalities in mitochondrial functioning, whether this is congenital or acquired or a combination of these is still conjectural [119].
Despite guidance from various government drug agencies contraindicating its use in children following an unpublished study trialing 1 and 2 % propofol formulations versus conventional therapy [120, 121], propofol continues to be used in children but mostly with the aforementioned constraints and with a degree of caution [12]; but there is still no established consensus about what constitutes the safest dosage and under what circumstances [122].
After emergence of sporadic but serious outcomes in children, there followed similar reports in adults [123–125] even with relatively short infusions [126]. In a case report series, Cremer and colleagues identified adults who had head injuries but died with otherwise inexplicable cardiac arrests. This was attributed to the use of propofol with many of the associations of “propofol infusion syndrome” (metabolic acidosis, cardiac dysrhythmias, rhabdomyolysis, lipemia, and hyperkalemia) and it appeared to be associated with doses exceeding 5 mg kg−1 h−1 [127].
Further analysis of the electrocardiograms (ECGs) of these patients showed that they had developed ST segment elevation in leads V1 to V3 preceding the tachydysrhythmias that led to their deaths. The appearance of the ECG was similar to that found in Brugada syndrome and thus they speculated that PRIS could lead to an acquired version of this [128]. These ECG changes had also been noted elsewhere in young adults [129]. Despite recommendations regarding maximum propofol dosage rates [130], monitoring of the ECG, metabolic and biochemical markers, case reports of deaths in young adults still appear that describe a very similar picture [131].
In regard to the titratability of propofol to sedative effect, when formally studied in a randomized trial in children after cardiac surgery, there seemed to be some correlation between plasma levels and a derived EEG measure but not with clinical assessment of sedation status as measured by the COMFORT score. It was also noted that there was unpredictable variation in plasma levels with steady-state infusion rates [132].
Immunity
It has long been known that opioids affect the integrity of the immune system, but, arguably, the exact mechanism how this occurs is not yet fully elucidated. Possible mechanisms postulated are direct receptor-mediated effects on immune cells, via the hypothalamic–pituitary–adrenal axis or a combination of these [133].
Benzodiazepines have also been implicated in immunomodulatory effects. This can be mediated through inhibition of mast cells and reduction of pro-inflammatory mediator release and this appears also to be accounted for by direct binding sites on the walls of these cells [134].
Lymphocyte proliferation is diminished by morphine [135], thiopental, and midazolam, but appears to be well preserved by diazepam [136].
Propofol and midazolam were compared in terms of their effects on cytokine production in surgical ICU patients. Propofol was associated with higher levels of IL-1β(beta), IFN-γ(gamma), IL-6, and TNF-α(alpha), while midazolam was associated with decreases in these. IL-8 decreased in the presence of both agents, more with propofol. IL-2 also decreased with propofol. Some of these mediators are required for intact immune response rather than simply being an expression of inflammation, notably IFN-γ(gamma) and IL-2. Overall, it was felt that midazolam had less effect on the pro-inflammatory cytokines [137]. In an equine model, midazolam decreased phagocytosis and oxidative burst of neutrophils and macrophages [138].
Propofol inhibits human neutrophil chemotaxis, phagocytosis, and oxidative burst at clinically relevant blood concentrations. It is thought that this may be due to the inhibition of calcium influx into the cell [139]. These three aspects of neutrophil function were also depressed with clinically relevant concentrations of thiopental and midazolam while ketamine only affected phagocytosis [140].
Of relevance to the wider clinical usage of the alpha-2 agonists dexmedetomidine and clonidine, these two agents, by contrast, do not seem to affect neutrophil chemotaxis, phagocytosis, or superoxide production at clinically relevant concentrations [141].
Findings such as these have obvious potential implications for the use of such agents in the treatment of sepsis and systemic inflammation in the ICU.
Neuropathologic Effects
Considerable attention is currently being given to the role sedative, analgesic, and anesthetic agents may have on the developing brain. There is concern that apoptotic neurodegeneration may occur when exposed to certain agents. Volatile agents, midazolam and ketamine, have all been associated with apoptosis in immature rodents. However, doses studied in these experiments are often far in excess of those used in clinical human practice and in models of questionable analogy to pediatric anesthesia and intensive care [142]. A large prospective clinical study of preterm human infants (“EPIPAGE”) failed to show any deleterious effects associated with the prolonged use of sedative and analgesia in the intensive care of preterm infants [143]. Indeed under certain circumstances, some agents are thought to have neuroprotective properties (e.g., xenon, sevoflurane, ketamine, clonidine, and dexmedetomidine) particularly where some other insult such as hypoxic injury may preexist or be imminent [142]. Untreated pain seems to lead to apoptosis too, and this can be offset by the use of ketamine [144]. There is also data that suggest that undertreatment of noxious stimuli, at least in the preterm infant, is associated with a range of persisting symptoms in the older child such as lowered pain thresholds and behavioral and emotional problems [17].
The overall situation, therefore, is rather unclear at present.
Another area of concern is the effect that sedation may play in the neuromyopathy seen in intensive care patients. Two phenotypes are currently identified: polyneuropathy and myopathy [145]. Electrophysiology is helpful in distinguishing between the two, but both may occur simultaneously in the same patient. In some cases, therefore, measurement of serum creatine kinase and even muscle biopsies may help with diagnosis where atrophy or pan-fascicular necrosis may be observed [146, 147].
As regards polyneuropathy, no single causative process has been identified. Putatively it has been suggested that such neuropathies are subject to degradation of the “blood–brain barrier”—or in this setting the blood–nerve barrier, much as other capillary beds lose integrity in inflammatory pathologies commonly seen in the ICU. Sepsis per se is associated with such polyneuropathic weakness. However, whereas axonal degeneration is seen, this is not associated with local histological evidence of inflammation, as is seen in Guillain–Barré Syndrome [148].
Myopathy is also seen where nerve conduction studies are otherwise normal. This seems to be associated with loss of myosin more than actin as “thick filament loss” [146]. Factors that lend themselves to producing muscle wasting include immobility (essentially diminution of neural stimulation and loss of antigravity activity), malnutrition, and hormonal factors, particularly the administration of glucocorticoids [148]; but emerging data suggests that inflammation, as in sepsis or systemic inflammation due to hypoxic and/or ischemic origins, disturbs muscle mitochondrial function [145]. There is no evidence that humoral factors are at play—at least those that might be expected to be blocked by the administration of immunoglobulins (if the model of Guillain–Barré were to be mirrored) [148].
The use of muscle relaxants reinforces the deafferentation of skeletal muscle thus exacerbating the tendency to lose the trophic stimulus of neural activity [146, 148]. Other agents that potentiate the action of muscle relaxants, such as aminoglycoside antibiotics, will also contribute and there is evidence of synergy between glucocorticoids and muscle relaxants in this regard [146].
Overall, sedation contributes to the problem by the relative immobilization of the patient and reduction of skeletal activity. It is no accident that the time-honored exhortation to mobilize patients as soon as possible holds very true, but of course this has to be balanced against the sheer practicalities of achieving this in very sick patients.
There is no specific therapy to avoid such neuropathies and myopathies once they have occurred, but diagnosis of these conditions is helpful in appreciating what the patient is up against in terms of weaning from the ventilator and also mobilization. Recovery may take up to several months [145].
Measuring Sedation
There are several clinical scoring scales reported in the literature, but until relatively recently few that have been rigorously assessed for suitability in the pediatric ICU population. (Refer to Chaps. 5, 16, 17, and 20.) The adult ICU community is now facing a rationalization of the various sedation scales available in the literature. A recent review has identified 11 such scales and then subjected these to psychometric analysis [149]. Two emerged with the most favorable profiles: the Richmond Agitation-Sedation Scale [150] and the Sedation-Agitation Scale [151, 152].
The COMFORT score has been widely applied in pediatric sedation research literature. Developed in 1992, it consists of an eight-domain scale based on observations of: spontaneous movement, calmness, facial tension, alertness, respiratory activity, muscle tone, heart rate, and blood pressure [153]. Each domain could be scored from 1 to 5; a score of 17–26 was associated with satisfactory sedation, neither too deep nor light [154]. However, subsequent studies showed that the “physiological” variables, heart rate and blood pressure did not play a significant part [155, 156]. As a result, the abbreviated version—the “behavioral” COMFORT-B using the 6 remaining domains—has been widely adopted and validated [157].
The Hartwig scale was developed in a study examining sedation in neonates and infants and consists of five domains, each scored 1–5 for motor activity, grimacing, eye opening, and respiratory activity, but the last domain is dependent on tracheal suctioning [158]. It has been validated in the neonate and under-1-year-old infant age group [159].
The State Behavioral Scale (SBS) uses a different method of incremental stimulation, commencing with observation, then voice, then noxious stimuli—either planned tracheal suctioning or <5 s of nail bed pressure [5]. Essentially five parameters are examined: movement, calmness, alertness, response to stimulus, cough (spontaneous or to stimulation), respiratory drive/coordination with ventilator. Scores are then given as +2 to −3, in line with the respective broader group headings: agitated, restless/inconsolable, awake/calmable, response to voice or touch, response to noxious stimuli, unresponsive.
The University of Michigan Sedation Scale was developed expressly for assessing sedation in children undergoing procedures such as computed tomography scans [102]. It consists of a five-point scale from 0 (awake and alert) to 4 (unarousable), with the intervening stages being judged against voice, touch, or “significant” physical stimulation. An advantage is simplicity but it must be remembered that it only assesses conscious level without any other corroborative information and was only designed for short procedures without intubation and ventilation. It appears to correlate poorly with bispectral index scoring (BIS) for such outpatient procedures [160].
The Richmond Agitation-Sedation Scale (RASS) was developed in adult ICU [150] but also appears in PICU delirium-related literature [41]. It consists of a ten-point scale from “combative” at +4, then down in stages through 0 for “alert and calm,” and then further down to −5 for increasing sedation to “unrousable.” It also uses incremental stimulation, starting at observation, voice, and then shaking shoulder, then sternal rub.
It has been hoped that a more objective method of assessing the sedation level of patients might be forthcoming on the ICU, rather than relying on “just” clinical observer assessment and scoring.
Bispectral index (BIS) was originally developed as a “measure” of anesthesia and has an empirical, derived output of 0–100 from mainly low-frequency EEG signals, where a measure of <40 is associated with deep sedation and >80 may be associated with recall [161]. It has been observed that BIS scoring differs between age groups due to the different spread of electrophysiologic frequencies. When BIS is measured at uniform sedative doses, the output varies with age when infants and older children are compared and that arousability to a uniform stimulus at a given BIS level also differs similarly [162].
BIS has been studied repeatedly in the PICU but, on balance, has yet to prove itself reliable with all patients, with all drugs, and in all circumstances [163]. Triltsch found that there was correlation between COMFORT scores and BIS, but that occurred at deeper levels of sedation but not so much in the “target” area of COMFORT scores of 17–26. Additionally a BIS score of 83 seemed to delineate between light and deeper sedation [163]. It may have a role in detecting deep sedation and assessing sedation under muscle relaxation [161, 165, 166].
Courtman found that BIS had moderate correlation to the COMFORT score but that it was only able to discriminate between light and deep sedation, but recognized its potential in children under muscle relaxation [167]. Others also found both the Ramsay Score and COMFORT behavioral scale correlated reasonably with BIS in the unparalyzed child [161, 165].
In older BIS models there may have been cross talk between electromyographic (EMG) signals and those from the EEG as changes in the BIS signal were noted with the administration of muscle relaxants [168]; however, more recent versions of BIS (“BIS-XP”) have EMG compensation, although it is not clear to what extent this may alter how BIS readings correlate with levels of sedation [169].
Interestingly but importantly, when Froom and colleagues used twin BIS monitors in children on each side of the forehead, they found a discrepancy during stimulation. When they compared BIS to COMFORT scores, there was good correlation between COMFORT, mean BIS, and right and left BIS during light and moderate sedation; but during stimulation, the right BIS and COMFORT score did not correlate [170].
SNAP II Index is a more recent addition. This uses both low- and high-frequency electro-encephalogical (EEG) signals and is also expressed as an empiric scale 0–100. Previous experience has suggested that 50–65 was recommended for anesthesia. When this was studied in a PICU and compared to the COMFORT scores, a good correlation with COMFORT scoring was observed, particularly toward the deeper levels of sedation [171], though it is probably too early to draw any conclusions about an optimum SNAP II Index range. Previous work measuring a derived summed ratio (SR) of the EEG and comparing this with the COMFORT score during a staged emergence from propofol on the PICU showed that, with this agent at least, there was correlation with the SR, plasma levels of propofol, and the infusion rate, but the COMFORT score was a poor predictor of emergence—the children appearing to transition from deep sleep to very light sedation quite suddenly [132].
Delirium
There is an increasing appreciation of the role delirium plays in the management of the critically ill patient and the roles that primary pathologies and sedative practices play in this. Delirium is a disturbance of consciousness and cognition affecting the abilities to receive and process, store, and recall information. It is characterized by fluctuant symptoms, disorientation, hallucinations, and dysphoric elements such as fear, anger, or apathy and has been characterized as being either hyperactive (agitated) or hypoactive (lethargic). It is variously reported that the incidence of delirium in critically ill patients may be as high as 80 % in the more elderly populations in adult ICUs, at least at some point during an individual’s ICU admission [172]. When reviewing research findings in the literature, it is clear that differences occur between languages and some attempt has been made to clarify terminology to facilitate sharing of research [173]. In a recent prospective study in adults, the incidence of delirium was an independent risk factor associated with higher hospital mortality and 6-month follow-up mortality and less favorable level of functioning after hospital discharge [174].
What is the cause of this delirium? Almost certainly this is multifactorial, consequent to the varied challenges, pharmacological and pathophysiological, to normal brain physiology.
In a study of adult critically ill patients on mechanical ventilation and receiving intravenous sedation, polysomnography with respect to rapid eye movement (REM) sleep was studied in concert with circadian rhythmicity as evidenced by hourly urine excretion of melatonin metabolites (6-sulphatoxymelatonin or “aMT6s”). Severe disorganization of sleep–wake regulation and circadian activity was observed [175]. Recently, it has been found that, in a series of patients undergoing noncardiac surgery followed by a period of mechanical ventilation in the ICU, delirium was observed in those patients whose melatonin levels dropped in the first hour following their surgery—but was not related to subsequent melatonin levels [176].
Hypothetically at least, there is the possibility that some aspects of delirium may be caused by disruption of normal signaling between a circuit made up of the posterior parietal cortex, the medial temporal lobe, and the prefrontal cortex. The integrity of this “circuit” is important for normal cognition. It is activated and maintained by the ascending reticular activating system. Disturbances in this may have knock-on effects at higher cognitive levels resulting in the disturbances in orientation and affect seen in delirium [177]. Additionally, fluctuant levels of sedation, as measured by the Richmond Agitation-Sedation Scale, were associated with a greater risk of developing delirium [178].
Clearly, it is helpful to have a structured and validated assessment tool and this was achieved over 10 years ago in adult practice with the Confusion Assessment Method for the ICU (“CAM-ICU”) [172, 179]. In a recent survey of UK adult units, only 25 % screened for delirium. Of these, 55 % used a validated screening tool and of these, the majority (80 %) used the CAM-ICU [180].
S100B is a protein released into the blood in association with neurological injury. Routsi and colleagues showed that in adult patients without a primary neurological insult, over two-thirds of patients in their ICU had an elevated S100B level [181]. This was associated with elevated lactate levels, hemoglobin below 7 mg dL−1, and decreased pH and mean arterial blood pressure. As delirium occurs with a similar incidence, the question arises if both phenomena—one laboratory, the other clinical—may reflect the same underlying pathologies [182].
When examining the potential role of the neurotransmitters serotonin, dopamine, norepinephrine, and acetylcholine and their precursors (phenylalanine, tryptophan, and tyrosine), it was found that high and low ratios of both tryptophan and tyrosine with respect to other large neutral amino acids were related to the transitioning into delirium. This transitioning, in the same study, was also found to be related to higher severity of illness scores (Acute Physiology and Chronic Health Evaluation II [APACHE II]) and exposure to fentanyl [183].
How may delirium be prevented and treated? In the recent UK survey of adult ICUs, the most common “rescue” treatment was haloperidol and the most common second-line treatment was benzodiazepines, particularly with hyperactive delirium; but, in hypoactive delirium, the most common approach was not to add in any further drugs, though some haloperidol was used [180].
It is worth questioning whether benzodiazepines are helpful or in fact may be part of the problem. In a prospective, randomized, double-blind study comparing midazolam against dexmedetomidine, equivalent levels of sedation were achieved, but with reduced incidence of delirium (and reduced ventilation times) in the dexmedetomidine group [90]. It has also been shown that lorazepam can be an independent risk factor for the development of delirium [40]. In a comparison of dexmedetomidine and lorazepam in adult ICU patients with or without sepsis, it was found that the septic group randomized to dexmedetomidine fared better with respect to less days ventilated, less days exhibiting brain dysfunction, and decreased mortality [184].
Such analyses of the situation affecting children are, at present, less well advanced, though delirium on emergence from anesthesia has been recognized for some years and a validated scoring system was developed by Sikich and Lerman in 2004, the “PAED Scale” [185]. More recently, some pioneering work is now forthcoming in addressing the evaluation of delirium in pediatric ICU patients [41, 186]. While the undercurrent etiologies may be quite similar, diagnostic methodology will differ in relation to age-related differences in brain and intellectual functions [41], although the broad principles remain the same [186]. In 2007, Schieveld described a 6-year series of PICU patients with an overall incidence of delirium of 5 %, where this was categorized into 25 % hypoactive, 35 % hyperactive, but with the largest group “emerging” or “veiled” in 40 % [187]. This latter category was characterized by anxiety, moaning, and/or restlessness. In all groups, treatment was mostly with haloperidol, but about 25 % with risperidone. In contrast to adult practice, pediatric delirium has a higher incidence of the more agitated version and this has been borne out in other work [186]. Since then scoring of pediatric delirium has been refined with the use of the Delirium Rating Scale [188] and, particularly regarding the PICU setting, the introduction of a validated pediatric version of the CAM-ICU, the “pCAM-ICU” [41].
There is a recent publication of guidelines by the American College of Critical Care Medicine for the management of pain, agitation, and delirium in adult ICU patients [189], but these are related to adult practice and further elucidation of delirium in the pediatric population will hopefully lead to similar guidelines. However, it is noteworthy that when previously the use of haloperidol had been suggested as an agent that might reduce the duration of delirium, this has now been dropped from the most recent version of these guidelines [189] and a recent study in adults seems to confirm lack of benefit of haloperidol in reducing delirium incidence or duration [190]. There is some interest, therefore, in finding other strategies or agents that may prevent or at least deal with delirium in ICU patients. In adult delirium patients, where rivastigmine had been shown to have some benefit in treating non-ICU patients, when this was examined in ICU patients, there was no apparent benefit and possibly some harm [191]. Presumably therefore, and perhaps unsurprisingly, there must be other confounding factors at play in what intensive care entails that complicates the etiology of delirium.
Tolerance and Withdrawal
There are a number of terms in this area of practice, some of which have overlapping and interrelated elements: tolerance, tachyphylaxis, addiction, dependence, and withdrawal.
Anand has attempted to delineate these [28]: addiction is characterized by psychological dependence and is a chronic and often relapsing disorder, rarely a facet of PICU practice. Tolerance and tachyphylaxis are, arguably, separated by their time course. Tachyphylaxis is seen with rapid loss of drug effects, e.g., with exhaustion of transmitters or activation of antagonistic systems such as the NMDA receptors. Tolerance is associated with more prolonged exposure and may reflect desensitization of receptors or up-regulation of compensatory intracellular processes (e.g., the cAMP pathway with opioid use [27]). Dependence is the physiological and biochemical adaptation of neurons where removal of a drug precipitates withdrawal or “abstinence” symptoms and signs. Withdrawal is a clinical syndrome of varied clinical symptoms that occurs after stopping a drug after prolonged exposure and where a form of dependence has been caused by adaptive physiology as outlined above.
Whereas tolerance and the reverse side of the coin, withdrawal, have long been associated with the use of opioids [28, 192], it has taken longer to appreciate that other agents used in the field of sedation and analgesia are capable of inducing tolerance and withdrawal [61, 192–194], including propofol [37, 195], midazolam [12, 42, 196, 197], isoflurane [61], clonidine [198], and dexmedetomidine [104, 105, 109].
Opioid tolerance appears to occur earlier in younger patients, perhaps exacerbated by background neurological insult [199], and with the shorter acting forms with high affinity for opioid receptors [28, 30, 200, 201] and this can occur quite rapidly, even during the course of anesthesia [29].
Benzodiazepine withdrawal seems to be associated with higher total doses, particularly regarding midazolam [12, 42]. However, these larger doses may reflect the underlying degree or nature of the illness affecting the child itself [12]. Additionally, these series were reported without cognizance of any underlying delirium—another emerging complication that needs to be considered and that, arguably, may have been present at the time [202].
Until recently, the assessment of withdrawal in children has been hampered by the lack of a tool constructed expressly for the pediatric ICU population. Historically, the literature in this area frequently utilized the Neonatal Abstinence Score. However, this was only devised to assess symptoms in neonates born to mothers suffering from addictions [203]. An adaptation of this, aimed at assessing children outside the infant group, the Sedation Withdrawal Score was described by Cunliffe. It compromised 12 clinical features, each scored from 0 to 2 (absent, mild, severe), giving a possible range of 0–24 [196].
Ista and colleagues described an array of 24 symptoms that were possibly associated with withdrawal in a pediatric ICU population, signposting the direction of future efforts at finding a suitable validated and reproducible tool [204].
Two validated scoring tools are now available: the Withdrawal Assessment Tool-1 (WAT-1) [6] and the Sophia Observation Score (SOS) [205]. Arguably the WAT-1 is more adept at picking up withdrawal from opioids than benzodiazepines [6, 205]. The WAT-1 consists of 11 domains: presence of loose stools, vomiting/gagging, pyrexia >37.8 °C, observation of “State” using the State Observation Scale [5], tremor, sweating, abnormal movements, yawning/sneezing, startle to touch, muscle tone, and time to regain calm after stimulus. The test needs about 7 min for completion and gives a possible score range of 0–12, with high scores indicative of the extent of withdrawal with a score of >3 reflective of length of stay, necessity to wean of sedation, duration of mechanical ventilation, and ICU stay. These findings were confirmed by a recent validation exercise [206].
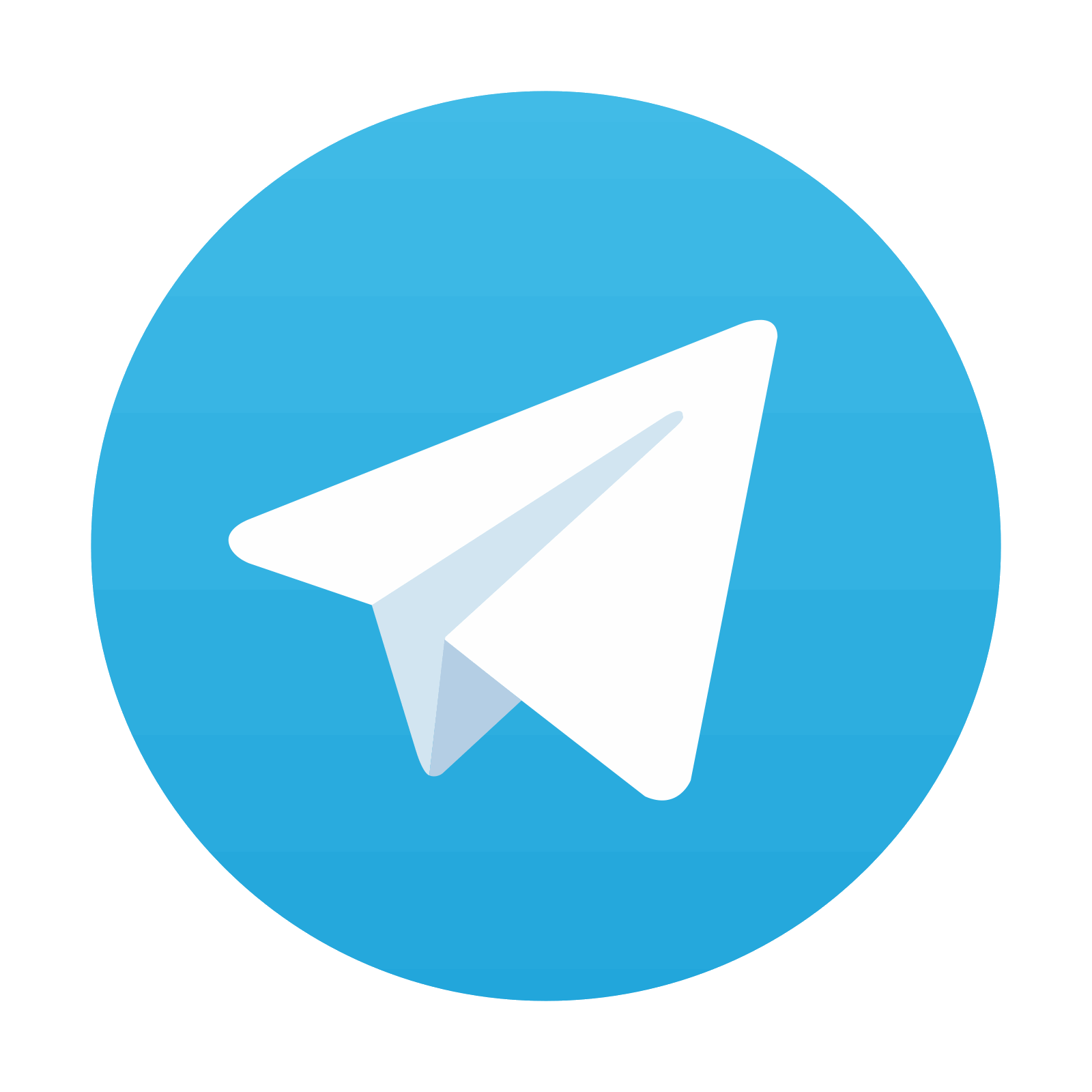
Stay updated, free articles. Join our Telegram channel
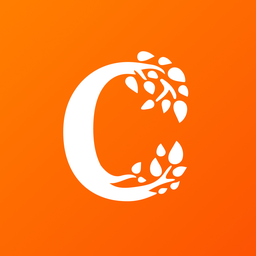
Full access? Get Clinical Tree
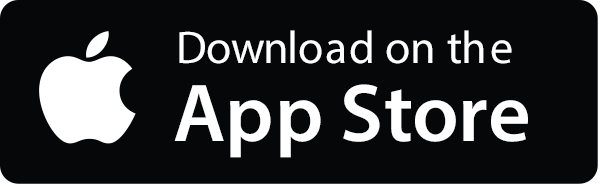
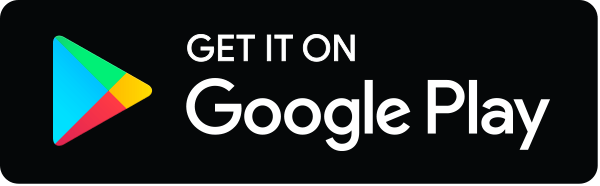