Chapter 65 Respiratory Distress and Failure
The term respiratory distress is often used to indicate signs and symptoms of abnormal respiratory pattern. A child with nasal flaring, tachypnea, chest wall retractions, stridor, grunting, dyspnea, and wheezing is often judged as having respiratory distress. The magnitude of these findings is used to judge the clinical severity of respiratory distress. Although nasal flaring is a nonspecific sign, the other signs may be useful in localizing the site of pathology (Chapter 365). Respiratory failure is defined as inability of the lungs to provide sufficient oxygen (hypoxic respiratory failure) or remove carbon dioxide (ventilatory failure) to meet metabolic demands. Whereas respiratory distress is a clinical impression, the diagnosis of respiratory failure indicates inadequacy of oxygenation or ventilation or both. Respiratory distress can occur in patients without respiratory disease, and respiratory failure can occur in patients without respiratory distress.
Respiratory Distress
Nasal flaring is an extremely important sign of distress, especially in infants. It is indicative of discomfort, pain, fatigue, or breathing difficulty. The state of responsiveness is another crucial sign. Lethargy, disinterest in surroundings, and poor cry are suggestive of exhaustion, hypercarbia, and impending respiratory failure. Abnormalities of the rate and depth of respirations can occur with both pulmonary and nonpulmonary causes of respiratory distress. In diseases of decreased lung compliance, such as pneumonia and pulmonary edema, respirations are characteristically rapid and shallow (decreased tidal volume). In obstructive airway diseases, such as asthma and laryngotracheitis, respirations are deep (increased tidal volume) but less rapid. Rapid and deep respirations without other respiratory signs should alert the physician to the possibility of nonrespiratory causes of respiratory distress, such as response to metabolic acidosis (diabetic ketoacidosis, renal tubular acidosis) or stimulation of the respiratory center (encephalitis, ingestion of central nervous system [CNS] stimulants). Chest wall, suprasternal, and subcostal retractions are manifestations of increased inspiratory effort, weak chest wall, or both. Inspiratory stridor indicates airway obstruction above the thoracic inlet, whereas expiratory wheezing results from airway obstruction below the thoracic inlet. Grunting is most commonly heard in diseases with decreased functional residual capacity (e.g., pneumonia, pulmonary edema) and peripheral airway obstruction (e.g., bronchiolitis).
Respiratory Disease Manifesting as Respiratory Distress
Clinical examination is important in localizing the site of pathology (Chapter 365). Extrathoracic airway obstruction occurs anywhere above the thoracic inlet. Inspiratory stridor, suprasternal, chest wall, and subcostal retractions, and prolongation of inspiration are hallmarks of extrathoracic airway obstruction. By comparison, features of intrathoracic airway obstruction are prolongation of expiration and expiratory wheezing. Typical manifestations of alveolar interstitial pathology are rapid, shallow respirations, chest wall retractions, and grunting. The site of pathology can be localized and the differential diagnosis established on the basis of the clinical signs and symptoms (Tables 65-1 and 65-2).
Table 65-2 EXAMPLES OF ANATOMIC SITES OF LESIONS CAUSING RESPIRATORY FAILURE
LUNG | RESPIRATORY PUMP |
---|---|
CENTRAL AIRWAY OBSTRUCTION | THORACIC CAGE |
PERIPHERAL AIRWAY OBSTRUCTION | BRAINSTEM |
Alveolar-Interstitial Disease | Spinal Cord |
NEUROMUSCULAR | |
ARDS, acute respiratory distress syndrome; CNS, central nervous system.
Respiratory Distress without Respiratory Disease
Although respiratory distress most commonly results from diseases of lungs, airways, and chest wall, pathology in other organ systems can manifest as “respiratory distress” and lead to misdiagnosis and inappropriate management (Table 65-3). Respiratory distress resulting from heart failure or diabetic ketoacidosis may be misdiagnosed as asthma and improperly treated with albuterol, resulting in worsened hemodynamic state or ketoacidosis.
Table 65-3 NONPULMONARY CAUSES OF RESPIRATORY DISTRESS
Cardiovascular Disease Manifesting as Respiratory Distress
A child with cardiovascular pathology may present with respiratory distress caused by 2 mechanisms: (1) decreased lung compliance and (2) cardiogenic shock (Table 65-4). Diseases that result in an increased pulmonary arterial blood flow (e.g., left-to-right shunts) or increased pulmonary venous pressure (e.g., left ventricular dysfunction from hypertension or myocarditis, obstructed total anomalous pulmonary venous return) cause an increase in pulmonary capillary pressure and transudation of fluid into the pulmonary interstitium and alveoli. The increased pulmonary blood and water content leads to decreased lung compliance and results in rapid shallow respirations.
Table 65-4 CARDIOVASCULAR PATHOLOGY MANIFESTING AS RESPIRATORY DISTRESS
Interstitial edema often results in small airway obstruction, manifesting as expiratory wheezing. Patients with cardiac lesions that result in a low cardiac output state, such as obstructive lesions of left side of the heart and acquired or congenital cardiomyopathy, often present in a state of shock with decreased tissue perfusion and metabolic acidosis. Such children demonstrate respiratory distress because of stimulation of chemoreceptors by metabolic acidosis and stimulation of baroreceptors by decreased blood pressure.
Neurologic Disease Manifesting as Respiratory Distress
CNS dysfunction can lead to alterations in respiratory patterns. Increased intracranial pressure (ICP) may manifest as respiratory distress. Early rise in ICP results in stimulation of respiratory centers, leading to increases in the rate (tachypnea) and depth (hyperpnea) of respiration. The resultant decrease in PaCO2 and elevation of cerebrospinal fluid pH lead to cerebral vasoconstriction and amelioration of intracranial hypertension. Cerebral hemispheric and midbrain lesions often result in hyperpnea as well as tachypnea. In such situations, blood gas measurements typically show respiratory alkalosis without hypoxemia. Pathology affecting the pons and medulla manifests as irregular breathing patterns such as apneustic breathing (prolonged inspiration with brief expiratory periods), Cheyne-Stokes breathing (alternate periods of rapid and slow breathing), and irregular, ineffective breathing or apnea. Level of consciousness is most often impaired when abnormal breathing pattern from a brainstem disorder is present. Along with respiratory changes, other manifestations of CNS dysfunction and increased ICP may be present, such as focal neurologic signs, pupillary changes, hypertension, and bradycardia (Chapter 63). Occasionally, severe CNS dysfunction can result in neurogenic pulmonary edema (NPE) and respiratory distress, which may be due to excessive sympathetic discharge resulting in increased pulmonary venous hydrostatic pressure as well as increased pulmonary capillary permeability. Central neurogenic hyperventilation is characteristically observed in CNS involvement by illnesses such as Reye syndrome and encephalitis. Bradycardia and apnea may be due to CNS-depressant medications, poisoning, prolonged hypoxia, trauma, or infection (see Table 65-2).
Toxic-Metabolic States Manifesting as Respiratory Distress
Direct stimulation of respiratory centers resulting in respiratory alkalosis is encountered in certain intoxications, such as those involving salicylates and theophylline. Similarly, intoxication with general CNS stimulants such as cocaine and amphetamines may manifest as increased respirations. Presence of endogenous and exogenous toxins, such as organic acidemias, ingestion of methanol and ethylene glycol, and late stages of salicylism, cause metabolic acidosis and compensatory hyperventilation, which can manifest as respiratory distress. Blood gas measurements show decreased pH and compensatory hypocarbia with normal oxygenation. Metabolic disorders causing hyperammonemia, on the other hand, cause respiratory alkalosis (decreased PaCO2 with increased pH) because ammonia is a stimulant of respiratory centers.
Other Nonpulmonary Entities Manifesting as Respiratory Distress
Sepsis and septic shock may manifest as respiratory distress by causing acute respiratory distress syndrome [ARDS], hypovolemic stimulation of baroreceptors, stimulation of respiratory centers by cytokines, and lactic acidosis. Similarly, renal disease may manifest as respiratory distress by causing metabolic acidosis (e.g., renal tubular acidosis or renal failure) or hypertensive left ventricular failure and fluid overload.
Respiratory Failure
Respiratory failure occurs when oxygenation and ventilation are insufficient to meet the metabolic demands of the body. Respiratory failure may result from an abnormality in (1) lung and airways, (2) chest wall and muscles of respiration, or (3) central and peripheral chemoreceptors (Fig. 65-1). Clinical manifestations depend largely on the site of pathology. Although respiratory failure is traditionally defined as respiratory dysfunction resulting in PaO2 < 60 torr with breathing of room air and PaCO2 > 50 torr resulting in acidosis, the patient’s general state, respiratory effort, and potential for impending exhaustion are more important indicators than blood gas values.
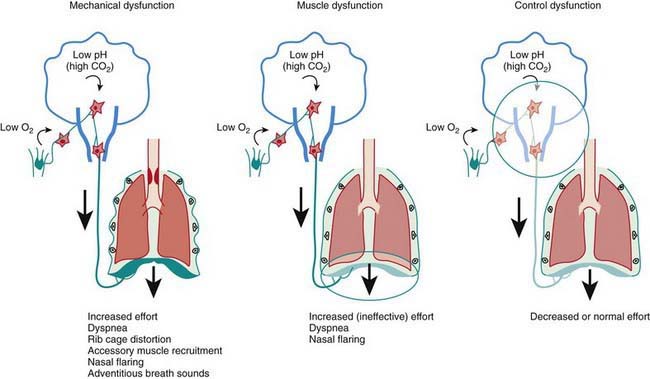
Figure 65-1 Presentation profiles of respiratory failure in childhood. When a mechanical dysfunction is present (by far, the most common circumstance), arterial hypoxemia and hypercapnia (and hence pH) are sensed by peripheral (carotid bodies) and central (medullary) chemoreceptors. After the information is integrated with other sensory information from the lungs and chest wall, chemoreceptor activation triggers an increase in the neural output to the respiratory muscles (vertical arrows), which results in the physical signs that characterize respiratory distress. When the problem resides with the respiratory muscles (or their innervation), the same increase in neural output occurs (arrow), but the respiratory muscles cannot increase their effort as demanded; therefore, the physical signs of distress are more subtle. Finally, when the control of breathing is itself affected by disease, the neural response to hypoxemia and hypercapnia is absent or blunted, and the gas exchange abnormalities are not accompanied by respiratory distress.
Acute lung injury due to pneumonia, sepsis, aspiration, drowning, embolism, trauma, smoke inhalation, or drug overdose often leads to the acute respiratory distress syndrome (Table 65-5; Fig. 65-2).
Table 65-5 SIMPLIFIED CONSENSUS DEFINITION OF ACUTE LUNG INJURY
From Wheeler AP, Bernard GR: Acute lung injury and the acute respiratory distress syndrome: a clinical review, Lancet 369:1553–1564, 2007.
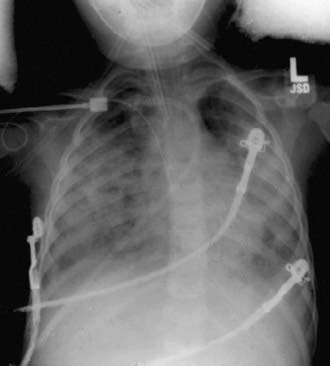
Figure 65-2 Frontal portal chest radiograph showing diffuse bilateral infiltrates consistent with acute lung injury.
(From Wheeler AP, Bernard GR: Acute lung injury and the acute respiratory distress syndrome: a clinical review, Lancet 369:1553–1564, 2007.)
Pathophysiology of Respiratory Failure
Respiratory failure can be classified into 2 categories: (1) hypoxic respiratory failure (failure of oxygenation) and (2) hypercarbic respiratory failure (failure of ventilation). The two entities may coexist as a combined failure of oxygenation and ventilation. The main function of the respiratory system is to move atmospheric gases into the alveolar capillary units of the lung and to move alveolar gas back out into the atmosphere. Systemic venous (pulmonary arterial) blood is arterialized after mixing with the alveolar gas and being carried back to the heart by pulmonary veins. The arterial gas composition depends on the gas composition of the atmosphere and the effectiveness of alveolar ventilation, pulmonary capillary perfusion, and diffusion across the alveolar capillary membrane. Abnormality at any of these steps can result in respiratory failure.
Inspired Gas Composition
Unless modified by the caretaker, inspired gas consists mainly of oxygen and nitrogen. The atmospheric pressure of inspired air depends on altitude. At sea level, it is 760 torr (mm Hg). At higher altitude, atmospheric pressure is lower; in Denver, for instance, the barometric pressure is around 630 torr. When the atmospheric gas reaches alveoli, it is 100% humidified. At 100% humidity and a temperature of 37°C, the water vapor pressure is 47 torr regardless of altitude. Therefore, pressure of inspired gases is calculated as barometric pressure minus 47 torr. Because the fraction of inspired oxygen (FIO2) is 0.2093 (close to 0.21) throughout our atmosphere, the PO2 of inspired gas (PIO2) is (760 − 47) × 0.21 = 150 torr in San Diego (sea level) and (630 − 47) × 0.21 = 122 torr in Denver. Lower PIO2 value at a higher altitude has a potentially adverse effect on oxygenation in respiratory disease. PIO2 can be increased by either an increase in FIO2 or administration of oxygen at greater than atmospheric pressure, such as in a hyperbaric chamber. For a person breathing 40% oxygen, the PIO2 would be 285 torr in San Diego and 234 torr in Denver.
Alveolar Gas Composition
When atmospheric gas enters alveoli, oxygen is exchanged for carbon dioxide. This exchange is not equimolar because the body’s average oxygen consumption is greater than carbon dioxide production, as represented by the respiratory quotient (R), which is CO2 production ÷ O2 consumption. Alveolar gas composition is roughly estimated by the following equation:
where PAO2 is the expected partial pressure of oxygen in alveoli. Under normal metabolic conditions, R is assumed to be 0.8. PAO2 is traditionally compared with PaO2 to determine the extent of oxygenation abnormality; this comparison is referred to as the alveolar-arterial oxygen gradient (A-aO2).
Alveolar Ventilation and Dead Space Ventilation
The amount of air breathed in a minute is termed minute volume, which is a product of tidal volume (VT) and respiratory rate. Part of the VT occupies conducting airways (anatomic dead space) and does not participate in gas exchange. Additionally, air that enters alveoli that are not perfused (e.g., from pulmonary embolism) or poorly perfused (e.g., because of decreased cardiac output) also does not contribute to gas exchange (physiologic dead space). Total dead space (VD) is a sum of anatomic and physiologic dead spaces. Alveolar ventilation (VA) therefore is calculated as follows:
At a constant level of CO2 production, PaCO2 is inversely proportional to alveolar ventilation. An increase in PaCO2 from 40 torr to 80 torr is indicative of a 50% decrease in VA, and a decrease in PaCO2 from 40 torr to 20 torr reflects doubling of VA. An elevated PaCO2, which is indicative of alveolar hypoventilation, can occur from airway obstruction, weakness of respiratory muscles, or CNS dysfunction (hypoventilation).
Ventilation-Perfusion Mismatch, Venous Admixture, Intrapulmonary Shunt
For exchange of O2 and CO2 to occur, alveolar gas must be exposed to blood in pulmonary capillaries. Both ventilation and perfusion are lower in nondependent areas of the lung and higher in dependent areas of the lung. The difference in perfusion () is greater than the difference in ventilation (
). Perfusion in excess of ventilation results in incomplete “arterialization” of systemic venous (pulmonary arterial) blood and is referred to as venous admixture. Perfusion of unventilated areas is referred to as intrapulmonary shunting of systemic venous blood to systemic arterial circulation. Conversely, ventilation that is in excess of perfusion is “wasted”; that is, it does not contribute to gas exchange and is referred to as dead space ventilation. Dead space ventilation results in return of greater amounts of atmospheric gas (which has not participated in gas exchange and has negligible CO2) to the atmosphere during exhalation. The end result is a decrease in mixed expired PCO2 (PECO2) and an increase in the PaCO2-PECO2 gradient. The fraction of tidal volume that occupies dead space (VD/VT) is calculated as follows:
Normal VD/VT is around 0.33. VD/VT increases in states that result in decreased pulmonary perfusion, such as pulmonary hypertension, hypovolemia, and decreased cardiac output. Venous admixture and intrapulmonary shunting predominantly affect oxygenation, resulting in a PAO2-PaO2 (A-aO2) gradient without elevation in PaCO2. The reason is the greater ventilation of perfused areas, which is sufficient to normalize PaCO2 but not PaO2 because of their respective dissociation curves (Chapter 365). The relative straight-line relationship of hemoglobin-CO2 dissociation allows for averaging of PCO2 from hyperventilated and hypoventilated areas. Because the association between oxygen tension and hemoglobin saturation plateaus with increasing PaO2, the decreased hemoglobin-O2 saturation in poorly ventilated areas cannot be compensated for by well-ventilated areas where hemoglobin-O2 saturation has already reached near-maximum. This results in decreased SaO2 and PaO2. Elevation of PaCO2 in such situations is indicative of attendant alveolar hypoventilation. Examples of diseases leading to venous admixture include asthma and aspiration pneumonia, and those of intrapulmonary shunt include lobar pneumonia and acute respiratory distress syndrome.
Diffusion
Even if ventilation and perfusion are matched, gas exchange requires diffusion across the interstitial space between alveoli and pulmonary capillaries. Under normal conditions, there is sufficient time for the pulmonary capillary blood to equilibrate with alveolar gas across the interstitial space. When the interstitial space is filled with inflammatory cells or fluid, diffusion is impaired. Because the diffusion capacity of CO2 is 20 times greater than that of O2, diffusion defects manifest as hypoxemia rather than hypercarbia. Even with the administration of 100% oxygen, PAO2 increases to around 660 torr from 100 torr at sea level, and the concentration gradient for diffusion of O2 is increased by only 6.6 times. Therefore, with diffusion defects, lethal hypoxemia will set in before clinically significant CO2 retention results. In fact, in such situations PCO2 is often decreased because of the hyperventilation that accompanies hypoxemia. Presence of hypercarbia in diseases that impair diffusion is indicative of alveolar hypoventilation from coexisting airway obstruction, exhaustion, or CNS depression. Examples of disease that impair diffusion are interstitial pneumonia, ARDS, scleroderma, and pulmonary lymphangiectasia.
Monitoring a Child in Respiratory Distress and Respiratory Failure
Clinical Examination
Clinical observation is the most important component of monitoring. The presence and magnitude of abnormal clinical findings, their progression with time, and their temporal relation to therapeutic interventions serve as guides to diagnosis and management (Chapter 365). The child with respiratory distress or failure should be observed in the position of greatest comfort and in the least threatening environment.
Pulse oximetry is the most commonly utilized technique to monitor oxygenation. Noninvasive and safe, it is the standard of care in bedside monitoring of children during transport, procedural sedation, surgery, and critical illness. It indirectly measures arterial hemoglobin-O2 saturation by differentiating oxyhemoglobin from deoxygenated hemoglobin using their respective light absorption at wavelengths of 660 nm (red) and 940 nm (infrared). A pulsatile circulation is required to enable detection of oxygenated blood entering the capillary bed. Percentage of oxyhemoglobin is reported as arterial oxyhemoglobin saturation (SaO2); however, the correct description is oxyhemoglobin saturation as measured by pulse oximetry (SpO2). This is because SpO2 may not reflect SaO2 in certain situations. It is important to be familiar with the hemoglobin-O2 dissociation curve (Chapter 365) in order to estimate PaO2 at a given oxyhemoglobin saturation. Because of the shape of the hemoglobin-O2 dissociation curve, changes in PaO2 above 70 torr are not readily identified by pulse oximetry. Also, at the same PaO2 level, there may be a significant change in SpO2 at a different blood pH value. In most situations, an SpO2 value greater than 95% is a reasonable goal, especially in emergency situations. There are exceptions, such as in patients with single ventricle cardiac lesions, in whom the pulmonary and systemic circulations are receiving blood flow from the same ventricle (e.g., after Norwood procedure for hypoplastic left heart syndrome), or with large left-to-right shunts (e.g., ventriculoseptal defect [VSD] and patent ductus arteriosus). In these types of pathophysiologic situations, a lower SpO2 is desired to avoid excessive blood flow to the lungs and pulmonary edema from the pulmonary vasodilatory effects of oxygen, and, in the patient with a single ventricle, diverting blood flow away from the systemic circulation. Because pulse oximetry recognizes all types of hemoglobin as either oxyhemoglobin or deoxygenated hemoglobin, it provides inaccurate information in the presence of carboxyhemoglobin and methemoglobin. Percentage of oxyhemoglobin is overestimated in carbon monoxide poisoning and methemoglobinemia. It should be recognized that dangerous levels of hypercarbia may exist in patients with ventilatory failure, who have satisfactory SpO2 if they are receiving supplemental oxygen. Pulse oximetry should not be the only monitoring method in patients with primary ventilatory failure, such as neuromuscular weakness and CNS depression. It is also unreliable in patients with poor perfusion and poor pulsatile flow to the extremities. Despite these limitations, pulse oximetry is a noninvasive, easily applicable, and effective means of evaluating the percentage of oxyhemoglobin in most patients.
Blood Gas Abnormalities in Respiratory Distress and Respiratory Failure
(See Chapters 52.7 and 365.)
Assessment of Oxygenation and Ventilation Deficits
Indicators for following clinical progress and for determining the prognosis in patients with defects in oxygenation or ventilation include:


Management
The goal of management for respiratory distress and respiratory failure is to ensure a patent airway and provide necessary support for adequate oxygenation of the blood and removal of CO2. Compared with hypercapnia, hypoxemia is a life-threatening condition, initial therapy for which should be aimed at ensuring adequate oxygenation.
Oxygen Administration
Supplemental oxygen administration is the least invasive and most easily tolerated therapy for hypoxemic respiratory failure. Nasal cannula oxygen provides low levels of oxygen supplementation and is easy to administer. Oxygen is humidified in a bubble humidifier and delivered via nasal prongs inserted in to the nares. In children, a flow rate <5 L/min is most often used because of increasing nasal irritation with higher rates. A common formula for an estimation of the FIO2 during use of a nasal cannula in older children and adults is as follows:
The typical FIO2 value using this method is between 23 and 40%, although the fraction of inspired oxygen varies according to the size of the child, the respiratory rate, and the volume of air moved with each breath. In a young child, because typical nasal cannula flows are a greater percentage of total minute ventilation, significantly higher FIO2 may be provided. Alternately, a simple mask may be employed, which consists of a mask with open side ports and a valveless oxygen source. Variable amounts of room air are entrained through the ports and around the side of the mask, depending on the fit, size, and minute volume of the child. Oxygen flow rates vary from 5 to 10 L/min, yielding typical FIO2 values between 0.30 and 0.65. If more precise delivery of oxygen is desired, other mask devices should be used.
A Venturi mask delivers preset fractions of oxygen through a mask and reservoir system by entraining precise amounts of room air into the reservoir with high-flow oxygen. The amount of room air entrainment and subsequent FIO2 are determined by the adapter at the end of each mask reservoir. The adapter can be chosen to provide between 30 and 50% oxygen concentrations. Oxygen flow rates of 5-10 L/min are recommended to achieve desired FIO2 and to prevent rebreathing. Partial rebreather and nonrebreather masks utilize a reservoir bag attached to a mask to provide higher fractions of oxygen. Partial rebreather masks have two open exhalation ports and contain a valveless oxygen reservoir bag. Some exhaled gas can mix with reservoir gas during exhalation, although most exits the mask via the exhalation ports. Through these ports, room air is also entrained during inspiration. A partial rebreather mask can provide up to 0.6 FIO2 as long as oxygen flow is adequate to keep the bag from collapsing (typically 10-15 L/min). As with nasal cannulas, smaller children with smaller tidal volumes entrain less room air, and their FIO2 values will be higher. Nonrebreather masks include two one-way valves, one between the oxygen reservoir bag and the mask and one on one of the two exhalation ports. This arrangement minimizes mixing of exhaled and fresh gas and entrainment of room air during inspiration. The second exhalation port has no valve, a safeguard to allow some room air to enter the mask in the event of disconnection from the oxygen source. A nonrebreather mask can provide up to 0.95 FIO2. The use of a nonrebreather mask in conjunction with an oxygen blender allows delivery of fractions of oxygen between 0.50 and 0.95. When supplemental oxygen alone is inadequate to improve oxygenation, or when ventilation problems coexist, additional therapies may be necessary.
Airway Adjuncts
Maintenance of a patent airway is a critical step in maintaining adequate oxygenation and ventilation. Artificial pharyngeal airways may be useful in patients with oropharyngeal or nasopharyngeal airway obstruction and in those with neuromuscular weakness in whom native extrathoracic airway resistance contributes to respiratory compromise. An oropharyngeal airway is a stiff plastic spacer with grooves along each side that can be placed in the mouth to run from the teeth along the tongue to its base just above the vallecula. The spacer prevents the tongue from opposing the posterior pharynx and occluding the airway. Because the tip sits at the base of the tongue, it is usually not tolerated by patients who are awake or whose gag reflex is strong. The nasopharyngeal airway, or nasal trumpet, is a flexible tube that can be inserted into the nose to run from the nasal opening along the top of the hard and soft palate with the tip ending in the hypopharynx. It is useful in bypassing obstruction from enlarged adenoids or from contact of the soft palate with the posterior nasopharynx. Because it is inserted past the adenoids, a nasopharyngeal airway should be used with caution in patients with bleeding tendencies.
Inhaled Gases
Helium-oxygen mixture (heliox) is useful in overcoming airway obstruction and improving ventilation. Helium is much less dense and slightly more viscous than nitrogen. When substituted for nitrogen, helium helps maintain laminar flow across an obstructed airway, decreases airway resistance, and improves ventilation. It is especially helpful in diseases of large airway obstruction in which turbulent airflow is more common, such as acute laryngotracheobronchitis, subglottic stenosis, and vascular ring. It is also used in patients with severe status asthmaticus. To be effective, helium should be administered in concentrations of at least 60%, so associated hypoxemia may limit its use in patients requiring more than 40% oxygen. Nitric oxide (NO) is a powerful inhaled pulmonary vasodilator. Its use may improve pulmonary blood flow and mismatch in patients with diseases that elevate pulmonary vascular resistance, such as persistent pulmonary hypertension of the newborn (PPHN), primary pulmonary hypertension, and secondary pulmonary hypertension due to chronic excess pulmonary blood flow (e.g., VSD) or collagen vascular diseases. NO is administered in doses ranging from 5 to 20 parts per million (ppm). Although administration of NO to unintubated patients is possible, it is usually administered to patients receiving mechanical ventilation through endotracheal tubes, because of the need for precision in NO dosing.
Positive Pressure Respiratory Support
Noninvasive positive pressure respiratory support is useful in treating both hypoxemic and hypoventilatory respiratory failure. Positive airway pressure helps aerate partially atelectatic or filled alveoli, prevent alveolar collapse at end exhalation, and increase functional residual capacity (FRC). This improves pulmonary compliance and hypoxemia and decreases intrapulmonary shunt. In addition, positive pressure ventilation is useful in preventing collapse of extrathoracic airways by maintaining positive airway pressure during inspiration. Improving compliance and overcoming airway resistance also improves tidal volume and therefore ventilation. A high-flow nasal cannula delivers gas flow at 4-16 L/min, providing significant continuous positive airway pressure (CPAP). The amount of CPAP provided is not quantifiable and varies with each patient, depending on the percentage of total inspiratory flow that is delivered from the cannula, airway anatomy, and degree of mouth breathing. In small children, the relative amount of CPAP for a given flow is usually greater than in older children and may provide significant positive pressure. The FIO2 can be adjusted by provision of gas flow through an oxygen blender. For delivery of high-flow air or oxygen, adequate humidification is essential and is achieved with use of a separate heated humidification chamber. CPAP can also be provided through snugly fitting nasal prongs or a tight-fitting facial mask attached to a ventilator or other positive pressure device. Noninvasive CPAP is most useful in diseases of mildly decreased lung compliance and low FRC, such as atelectasis and pneumonia. Diseases of extrathoracic airway obstruction in which extrathoracic negative airway pressures during inspiration lead to airway narrowing (e.g., laryngotracheitis, obstructive sleep apnea, postextubation airway edema) may also benefit from CPAP.
Bilevel positive airway pressure (BiPAP)
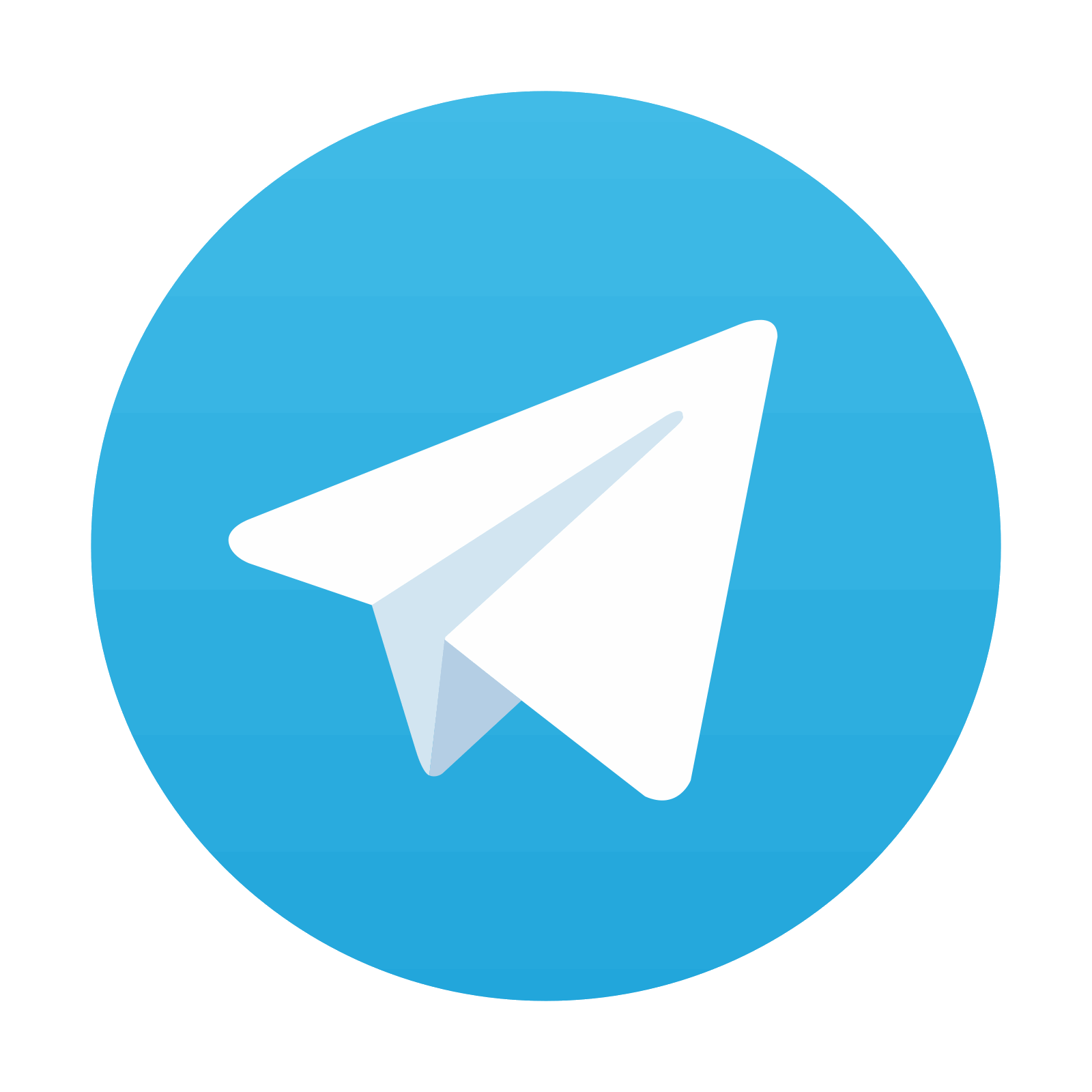
Stay updated, free articles. Join our Telegram channel
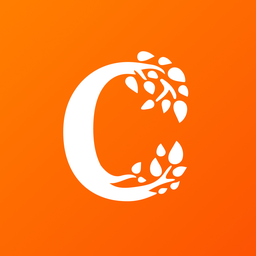
Full access? Get Clinical Tree
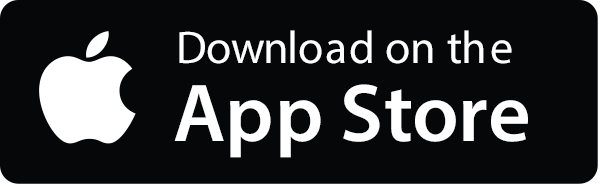
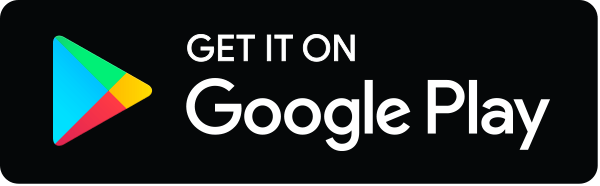