Group
Era
n
Dose (Gy)
5-year overall LC (%)
LC with RT alone
IESS-I
1973–1978
331
45–65
85
IESS-II
1978–1982
214
50–55
90
SJCRH
1978–1988
43
30–60
68
58
CESS-81
1981–1986
32
46–60
74
53
U of Fl
1982–1987
39
50–60
85
POG 8346
1983–1988
104
55.8
65
CESS-81
1986–1991
44
60
93
86
MSKCC
1990–2004
60
36–60
77a
7a
7.1.2 Indications for Radiotherapy
In a combined retrospective analysis of local therapy in the CESS 81 and 86 as well as the European Intergroup Ewing’s Sarcoma Study 92 (EICESS 92), Schuck et al. examined indications for radiation in 1,058 patients with non-metastatic Ewing tumor (Schuck et al. 2003). As in other studies, definitive radiotherapy was the preferred modality when surgery would only allow for intralesional resection or a debulking procedure. The primary criteria for post-operative radiation were resection status and poor pathologic tumor response. Not surprisingly, it was found that the addition of radiation improved local control in those with intralesional or marginal resections regardless of histologic response. However, patients deemed poor pathologic responders after wide resection experienced superior outcomes when treated with post-operative radiotherapy (local relapse of 5 % vs. 12 %), though the comparison is limited due to small patient numbers. Local control and EFS were equivalent in patients receiving radiation alone as compared with intralesional resection and adjuvant radiation, further solidifying the use of definitive radiation in poor surgical candidates. The EICESS 92 trial also permitted pre-operative radiotherapy in patients with <50 % reduction in the soft tissue tumor component. While there was no apparent improvement in EFS, the local relapse rate was only 5.3 % in this population, arguing for the use of neoadjuvant radiotherapy if function-preserving surgery with narrow margins is anticipated.
Current treatment guidelines call for post-operative radiation in the setting of residual gross or microscopic disease, and where there has been a suboptimal response to induction chemotherapy. This level of response has classically been defined as ≥10 % viable tumor cells in the surgical specimen. Based on the findings above some argue for the use of adjuvant radiotherapy, albeit to a lower total dose, in cases of gross total resection with adequate margins but a poor pathologic response (Donaldson 2004). This philosophy selects for a single “low risk” subgroup of patients who are precluded from radiotherapy due to both a satisfactory histologic response to chemotherapy and an appropriate margin status (>1 cm). Although variation in institutional practices exists, radiation is generally excluded when a wide resection has been achieved regardless of histologic response.
The appropriate timing for radiation delivery is not well defined, with prior studies initiating treatment anywhere from 9 to 18 weeks post-induction chemotherapy (Dunst et al. 1995; Schuck et al. 2003; Donaldson et al. 1998). Due to a number of confounding factors, it is difficult to determine how a potential delay in radiation may have affected long-term outcomes. Nonetheless it appears that a prolonged time to local therapy overall may lead to inferior outcomes due to possible interval development of drug resistance (Donaldson 2004). Regarding post-operative radiotherapy, European investigators found patients treated within a 60-day period following surgery experienced local control rates of 98 % versus 92 % for patients treated at later time points (Schuck et al. 2002). This difference was not statistically significant, nor did it influence survival. Current Children’s Oncology Group (COG) protocols generally require the incorporation of definitive radiotherapy when indicated at 12–13 weeks into systemic therapy, and 8 weeks post-surgical resection.
7.1.3 Volumes
For decades, radiation volumes for Ewing sarcoma encompassed the entire bone. This approach was prompted by several early reports implicating the propensity of Ewing tumors to spread along the medullary cavity of the bone (Phillips and Sheline 1969). A subsequent publication by Suit emphasized the rarity of local disease recurrence outside of the involved primary site (Suit 1964). This observation led to gradual reductions in field size with resultant equivalent local control. In the St. Jude Children’s Research Hospital experience, 94.7 % (18/19) of recurrences were confined to the irradiated bone (Arai et al. 1991). The radiation target volume was defined as the pre-chemotherapy osseous tumor in addition to the post-induction residual soft tissue extent with a 3 cm margin. The authors concluded that the lack of marginal failures indicated a reduction of irradiation volume was possible without incurring significant risk.
Similarly, the POG 8346 trial sought to determine if a more tailored radiation field was equivalent to standard whole bone radiation (Donaldson et al. 1998). Patients undergoing radiotherapy were prospectively randomized to either receive standard field treatment (SFRT), consisting of 39.6 Gy to the whole bone with a 16.2 Gy boost to the initial tumor plus a 2 cm margin, or involved field radiation (IFRT) to the initial tumor and a 2 cm margin with 55.8 Gy. Unfortunately the randomization portion of the study was discontinued prematurely due to poor accrual. However of the 40 patients randomized, there was no observed difference in 5-year EFS with SFRT versus IFRT (37 % vs. 39 % EFS). The remainder of the 94 patients received IFRT. The majority of local failures were central, within the radiotherapy port (62 %).
The precise definition of target volumes in radiation is crucial to successful execution. There are three main volumes in radiation planning. The gross tumor volume (GTV) consists of visible tumor present on physical exam and/or imaging. The second volume, the clinical target volume (CTV), contains the GTV plus a margin to include potential localized subclinical disease spread. The final treatment volume is known as the planning target volume (PTV). This is an expansion from the CTV to allow for uncertainties inherent in the technology, such as day-to-day minor variations in setup and patient movement.
Current radiotherapy contouring guidelines for Ewing sarcoma define GTV1 as the pre-treatment osseous and soft tissue disease (Donaldson 2004). In tumors that exhibit an infiltrative component into a body cavity (e.g., thorax, abdomen), the GTV1 may be modified to exclude the portion extending into the cavity if there has been a response to chemotherapy such that normal tissues have returned to their baseline position. A 1.5 cm margin is added to GTV1 to create CTV1. The expansion from CTV1 to PTV1 is institution specific, but typically is within the range of 0.5–1 cm.
For unresected tumors, GTV2 is the boost volume, and is defined as the pre-treatment boney abnormalities and residual soft tissue disease following induction chemotherapy. For inadequately resected tumors, GTV2 is the area of gross or microscopic residual disease, or the site of a close margin. A 1 cm margin is added to create the CTV2. Again, a 0.5–1 cm margin is added to the CTV2 to form the PTV2.
7.1.4 Dose and Fractionation
Given the high rate of in-field failure, studies have explored the utility of dose escalation. To date, the data is conflicting. The first Intergroup Ewing Sarcoma Study (IESS-I) did not demonstrate a dose response between 30 and 60 Gy (Razek et al. 1980). Local control was achieved in 6/6 (100 %) patients treated with 30–39.9 Gy, 37/43 (86 %) with 40–49.9 Gy, 80/91 (88 %) with 50–59.9 Gy, and 50/53 (94 %) with ≥60 Gy. Likewise, a retrospective review in an era of more modern radiation technique using 3D conformal and IMRT planning (see section on “Technique”) at Memorial Sloan Kettering Cancer Center (MSKCC) did not show a radiation dose response (Trang et al. 2006). Patients who received doses <55 Gy and ≥55 Gy experienced 3-year LC of 78 % and 73 %, respectively (p = 0.518).
Investigators at St. Jude Children’s Research Hospital found that lower doses of 30–36 Gy to tumors <8 cm in greatest dimension with an objective response to induction chemotherapy provided 5-year local control rate of 90 % (n = 9/10) (Arai et al. 1991). This rate was in comparison to a local control rate of 52 % (n = 11/21) in tumors ≥8 cm treated with identical doses. However, an update from the same institution by Krasin et al. showed higher rates of local recurrence in children with <8 cm tumors who received doses <40 Gy (19 % ± 9 %), with no local failures occurring with doses ≥40 Gy (Krasin et al. 2004). This difference approached statistical significance (p = 0.084). A recent paper published by Paulino et al. showed similar results. For tumors ≤8 cm, the 5-year local control rate was 50 % when treated with <49 Gy, versus 94.1 % when treated with doses ≥49 Gy. In tumors >8 cm, a radiation dose <54 Gy resulted in a 5-year local control rate of only 26.7 % compared to 85.7 % when treated with doses ≥54 Gy (Paulino et al. 2007a).
The recommended dose in definitive management of localized Ewing tumors is 55–60 Gy fractionated into 1.5–1.8 Gy doses. Such irradiation is typically delivered as 45 Gy to GTV1 with a 10.8 Gy boost to a reduced volume (GTV2) (Table 7.2). As suggested by Donaldson, a boost to a total dose of 60 Gy may be in order when there is <50 % soft tissue regression following induction chemotherapy (Donaldson 2004). In the adjuvant setting, gross residual disease or tumors with poor pathologic response should be treated to 55.8 Gy. Microscopic disease should receive a total dose of 50.4 Gy. Some institutions will also deliver 45 Gy to widely resected tumors with a poor response to chemotherapy (Donaldson 2004). It is important to note that substantial dose limitations exist depending upon the primary tumor site and adjacent critical strictures. For example, vertebral body tumors are traditionally treated to a maximal dose of 45 Gy with conventional fractionation due to the proximity of the spinal cord. Alternatively, the pre-chemotherapy osseous tumor and soft tissue disease plus a 2 cm margin can be treated to 45 Gy, and a boost dose to a total of 50.4 Gy can be given to the pre-chemotherapy osseous tumor and post-chemotherapy soft tissue extent with a 2 cm margin.
Table 7.2
Radiation recommendations
Setting |
Volume |
Margin (CTV + PTV) |
Dose (Gy) |
Total dose (Gy) |
---|---|---|---|---|
Definitive |
GTV1
GTV2 |
2–2.5 cm
1.5–2 cm |
45
10.8 |
55.8 |
Gross residual disease |
GTV1
GTV2 |
2–2.5 cm
1.5–2 cm |
45
10.8 |
55.8 |
Microscopic disease |
GTV1
GTV2 |
2–2.5 cm
1.5–2 cm |
45
5.4 |
50.4 |
≥10 % viable tumor cells |
55.8 |
55.8 |
When the heart is located within the treatment field for thoracic and chest wall lesions, the Children’s Oncology Group (COG) recommends that no more than 50 % of the heart be included, and that the maximum dose is ≤30.6 Gy. Similarly, 50 % of the liver should not receive >30 Gy. These normal tissue tolerance guidelines may require further modification in the presence of more intensive chemotherapeutic regimens.
The study of radiation dose is certainly crucial as late effects of radiotherapy have been documented with higher doses (Jentzsch et al. 1981; Paulino 2004; Kuttesch et al. 1996). One potential means of achieving a higher effective dose without the associated late morbidity is with an altered fractionation scheme. Hyperfractionation is the delivery of a total dose of radiation in a greater number of treatments (i.e., fractions), with a smaller dose per treatment. Accelerated fractionation refers to total dose delivery over a shorter time course, and serves to compensate for tumor repopulation that may occur when reducing the dose per fraction. Whereas conventional radiotherapy is typically given once daily, hyperfractionated regimens are generally delivered twice daily with an interval of at least 6 h between each treatment to reduce acute toxicity.
Many rapidly growing tumors remain sensitive to radiation even at lower fraction doses. Conversely, normal tissues that demonstrate a delayed response to radiation damage, also known as late-responding tissues (e.g., lung, kidney, spinal cord), are less sensitive to these smaller doses per fraction. Therefore accelerated hyperfractionation in certain malignancies can enhance the therapeutic ratio by reducing potential late effects while still allowing for optimal tumor control.
Historically, numerous Ewing’s sarcoma patients have been treated with accelerated hyperfractionation (Marcus et al. 1991; Bolek et al. 1996; Elomaa et al. 2000). Indelicato et al. proposed three theoretical advantages to this regimen (Indelicato et al. 2008). Firstly, the shortened treatment course allows for minimization of repopulation in a rapidly growing tumor. Secondly, Ewing sarcoma is a class of tumors (small round blue cell) that demonstrates high radiosensitivity; therefore, good cell kill is observed even with a smaller fraction size. Accelerated hyperfractionation schedules permit an increase in total dose in a given period of time and theoretically improve chances of local control. Finally it is well known that hyperfractionation can decrease late effects to nearby normal tissue. This final advantage serves as an undeniable benefit in the pediatric population.
Following the above premises, Italian SE-91 investigators are examining accelerated hyperfractionation as part of multimodal therapy in Ewing tumors, with the goal of improving overall and event-free survival (Rosito et al. 1999). The preliminary 3-year local control rate of 93–94 % with either definitive or adjuvant radiation is promising. However the data regarding utilization of conventional fractionation in comparison to a hyperfractionated regimen is mixed. In CESS 86, all patients treated with definitive or post-operative radiotherapy were randomized to either receive conventionally fractionated radiation alone or a hyperfractionated split course concurrent with systemic therapy (Dunst et al. 1995). The latter consisted of 1.6 Gy given twice daily with an interfraction interval of at least 6 h in two series of 22.4 Gy each when in an adjuvant setting. Definitive treatment involved two series of 22.4 Gy and a 16 Gy boost series. According to the authors, the primary objective of hyperfractionation was to administer radiation without an interruption in chemotherapy. Ultimately the type of fractionation (conventional vs. hyperfractionation) had no impact on local control (82 % vs. 86 %), relapse-free survival (53 % vs. 58 %), or overall survival (63 % vs. 65 %) in definitively treated patients. The same held true for post-operative cases. There was also no difference in the frequency of radiation-related complications amongst the two groups.
The University of Florida experience (Bolek et al. 1996; Indelicato et al. 2008) differed somewhat from these findings, as twice daily fractionation resulted in less range of motion loss, fibrosis, and limb length discrepancy. There was an increase in the likelihood of pathologic fracture in children treated with conventional fractionation as well, although this variation could be attributed to the comparatively larger field sizes and difference in radiation energies. Still, there was no statistically significant difference in 5-year local control rates between the two treatment groups. With these findings in mind, perhaps the most compelling reason for the use of hyperfractionation is the potential reduction in radiation-induced late sequelae.
7.1.5 Techniques: 3DCRT, IMRT, Proton/Ion
Radiation technique has made remarkable advancements over the years by incorporating improvements in imaging and computing power. The emergence of computed tomography (CT) and magnetic resonance imaging (MRI) has been particularly transformative, as all modern therapeutic radiation requires accurate delineation of not only the intended target volumes, but also the normal structures that must be avoided (McGovern and Mahajan 2012). External beam radiation (EBRT) refers to the delivery of radiation to a patient at a distance from an external source. Forms of EBRT vary through the application of diverse computer technologies. Traditionally, three-dimensional conformal radiotherapy (3DCRT) has been used in the treatment of Ewing’s sarcoma. This method incorporates computer assisted planning, allowing for field design and beam placement for an individual target based on diagnostic imaging. Normal tissue can additionally be strategically blocked using multi-leaf collimation (MLC) built into linear accelerators. Conversely, IMRT employs computer based inverse planning algorithms where MLCs are used to generate intensity maps within a given radiation field by integrating parameters set by the planner to meet the objectives of a given treatment plan.
Due to its high level of precision and conformity, IMRT is a technique that is widely applied in the adult population. Theoretically, IMRT would be especially advantageous in pediatric patients where the concomitant sparing of neighboring organs at risk is just as imperative as ensuring adequate dose to the tumor itself. Yet the history of IMRT use in children is markedly different than that of adults for a number of reasons. Among these are increased fraction time, necessity for exact immobilization, and fear for secondary malignancy induction due to low dose spillage in surrounding tissues (Hall and Wuu 2003; Hall 2006; Schneider et al. 2006). In a single institution review of IMRT use in select pediatric cases, Sterzing et al. concluded the benefits of optimal disease control outweighed the risk of possibly increased rates of secondary malignancies (Sterzing et al. 2009). This finding was particularly true in cases of re-irradiation, where treatment with 3DCRT would have undoubtedly led to unacceptable morbidity. Authors from MSKCC argue the actual probability of secondary malignancy with IMRT is unlikely, given the literature has shown radiation-induced tumors to be dose dependent (Trang et al. 2006). In a study comparing the two techniques, Mansur et al. actually found that overall peripheral dose was similar between 3DCRT and IMRT plans for five pediatric patients with malignancies of the brain and skull base (Mansur et al. 2007).
Reports on IMRT planning in Ewing’s sarcomas are rare. However the implementation of this technique may prove useful in treatment of pelvic tumors, where the close proximity of disease to the bowel, bladder, or rectum presents appreciable challenges to eradication with either radiation or surgery. This was the basis of an eight patient analysis completed by Mounessi and colleagues (Mounessi et al. 2013). All patients were diagnosed with a pelvic Ewing’s sarcoma, and underwent planning with both 3DCRT and IMRT techniques. Results demonstrated IMRT to be superior in terms of conformity, resulting in a decrease of normal tissue exposure to high dose levels. There was a trade-off to this normal tissue sparing, however, as the dose homogeneity was lower with IMRT due to prioritizing of PTV coverage and organ sparing. There were also statistically significant higher volumes of normal tissue receiving low doses (2 Gy) of radiation in IMRT plans compared to 3DCRT. The authors concluded the enhanced therapeutic ratio provided by IMRT outweighed the potential risk of secondary malignancy. A recent publication from MSKCC also examined the utility of IMRT dose painting in a small group of Ewing sarcoma patients with pulmonary metastasis. There were no local failures, and an overall decreased mean dose to the esophagus, heart, spinal cord, and liver when compared to conventional methods (Yang et al. 2013). Although the data surrounding IMRT use in Ewing tumors is currently limited, there clearly appears to be an emerging role for this technique in the management of pediatric malignancies.
Charged particle therapy (proton and carbon ion) has been gaining popularity in the past several years, and has specifically piqued the interest of clinicians practicing in the field of pediatric oncology. This is due to the ability of particle therapy to deposit maximum dose at a defined depth with a sharp fall-off – a phenomenon known as the Bragg peak. Consequently, the dose exiting the body is essentially eliminated. Furthermore, there is a decreased dose entering the body as compared with photons. The end result is a highly conformal coverage of the radiotherapy target, but decreased dose to all other structures (Halperin 2005). The potential benefits of these radiotherapy modalities are obvious in the pediatric population, where concerns for normal tissue exposure and late sequelae are high. Currently charged particle therapy is most commonly applied in treatment of spinal sarcomas, malignancies of the head and neck, or CNS disease (Delaney et al. 2009). Clinical outcome studies thus far have confirmed a reduction in radiation-related toxicities without sacrificing tumor control, but more long-term follow-up is needed in order to validate the alleged benefits of particle therapy (McGovern and Mahajan 2012).
7.1.6 Site-Specific Considerations
Certain precautions should be taken when treating Ewing tumors at specific sites in order to ensure safe and effective treatment that also attenuates the potential for toxicity. For example, avoiding circumferential irradiation in extremity lesions is necessary to reduce the likelihood of fibrosis and edema. Furthermore, the probability of limb length discrepancies and growth aberrations can be reduced with exclusion of at least one epiphyseal plate when possible. Distal extremity tumors located at the feet or hands may require the use of tissue compensation, such as water or rice baths, to ensure homogeneous coverage of the radiotherapy target. Another option to achieving dose homogeneity in distal extremity tumors is application of a single-photon beam incident on the contralateral surface of the hand or foot combined with an ipsilateral electron field (Halperin 2005).
Rib lesions, also known as Askin tumors, pose unique challenges, given their large size and frequent extension to the pleural surfaces. As mentioned previously, the radiation volume and dose may be limited by nearby organs such as the heart or liver. In patients with cytologically positive effusions, inclusion of the pleural cavity in the treatment volume is recommended due to the high likelihood of local relapse. In general, when the vertebral bodies are included in the treatment field, the entire width should be encompassed so as to prevent radiation-induced scoliosis.
Finally, immobilization techniques including vacuum bags or thermoplastic masks are crucial to daily reproducibility during treatment. The actual delivery of conformal radiation can be further augmented with the use of image guided radiotherapy, which corrects for interfraction setup variations through employment of in-room or on-board imaging techniques such as cone-beam CT.
7.1.7 Quality
Given routine use of 3DCRT and IMRT for Ewing sarcoma, accurate target delineation becomes exceedingly important. Such precision has been greatly facilitated by the integration of CT and MRI into treatment planning, so much so that current COG guidelines require at least one of these modalities be completed for all Ewing sarcoma patients at the time of diagnosis (Meyer et al. 2008). In terms of radiotherapy, the additional impact of central quality review cannot be overlooked. For example, in the CESS 81 trial poor outcomes in patients treated with either definitive or post-operative radiotherapy (local failure rates of 47 % and 20 %, respectively) were partly attributed to poor quality control; 90 % of patients with protocol deviations experienced local relapse (Dunst et al. 1991). This finding prompted investigators involved in CESS 86 to implement a central radiation review (Dunst et al. 1995). With improved radiotherapy quality control, there was considerable improvement in local control (local failure rates of 14 % and 5 % in definitive and post-operative cases, respectively). The improvement was particularly evident with pelvic lesions and large tumors (>100 mL), with the likelihood of local relapse on CESS 81 of 40 % and 36 %, respectively, versus 10 % and 7 % on CESS 86.
Overwhelming disparities in local control rates were also found in the POG 8346 trial based on deviations in target volumes or dose distribution (Donaldson et al. 1998). Patients with no protocol deviations had a local control rate of 80 %, whereas those with either minor or major deviations had local control rates of 48 % and 16 %, respectively.
7.1.8 Metastatic Disease
The tendency for Ewing sarcoma to metastasize early, often exclusively to the lungs, stimulated interest in the use of prophylactic pulmonary radiotherapy. A complicating factor with lung irradiation is that pulmonary parenchymal tolerance can be exceeded before reaching tumoricidal doses (Whelan et al. 2002). Therefore administering radiation at a time point when only subclinical disease is present would require lower doses (and subsequently cause less toxicity) to achieve the same level of pulmonary control. This thinking was the premise behind the incorporation of prophylactic bilateral whole lung irradiation (WLI) in IESS-I (Nesbit et al. 1981). Patients with localized disease were randomized to one of three adjuvant treatment arms: standard vincristine, actinomycin D, and cyclophosphamide (VAC) chemotherapy, VAC plus adriamycin (VACA), or VAC plus prophylactic WLI. Long-term follow-up demonstrated an increased frequency of pulmonary metastasis after prophylactic WLI (20 %) as compared to VACA (15 %, p = 0.35) (Nesbit et al. 1990). There was also a statistically significant 5-year relapse-free survival advantage with VACA (60 %) over VAC plus WLI (44 %, p < 0.05) and VAC alone (24 %, p < 0.001). A similar improvement in 5-year OS was demonstrated with VACA versus VAC plus WLI (65 % vs. 44 %, p = 0.001), suggesting that the addition of adriamycin was superior to adjuvant prophylactic WLI.
Despite its lack of utility in the prophylactic setting, WLI has found a role in treatment of patients who present with overt pulmonary metastasis at the time of diagnosis, as supported by a retrospective review of 30 patients on the CESS trials (Dunst et al. 1993). At the time of the analysis, nine of the ten patients treated with WLI were in complete remission. A strong dose response was also observed, with relapsed patients having received significantly lower doses (12–16 Gy) than complete responders (18–21 Gy; p = 0.028). The authors concluded bilateral lung irradiation improved survival in patients with pulmonary spread.
A survival benefit with WLI to 14–18 Gy in patients with disseminated Ewing tumors was also shown on the EICESS trial (Bolling et al. 2008). This improvement held true whether patients had isolated pulmonary involvement or combined pulmonary and osseous disease. Of course, these findings must be interpreted with caution as the selection of therapy was in a non-randomized setting with a small number of patients. Results from the COG’s recent prospective randomized trial comparing bilateral WLI to a high-dose chemotherapy regimen using busulfan and melphalan will help clarify this issue.
The use of radiotherapy to extra-pulmonary metastatic sites has not yet been as thoroughly examined. Paulino et al. reported promising results in their study of patients with metastatic disease who received not only local treatment of their primary tumor, but also radiation to areas of distant osseous spread (Paulino et al. 2013). Of the six long-term survivors, all patients had irradiation to all bony sites of involvement. Interestingly, on multivariate analysis, only the use of local therapy to the primary site was found to be prognostic of both overall and progression-free survival, thus highlighting the importance of aggressive treatment to the primary tumor even in metastatic cases.
Finally, radiation has been widely accepted as an effective means of palliation, including children and adolescents with disseminated Ewing sarcoma. Investigators from Duke University demonstrated an overall response rate of 84 % in patients treated with palliative doses (median dose of 30 Gy) to osseous, pulmonary, and intracranial disease (Koontz et al. 2006). In a population with an estimated median survival of 1–2 years, the ability to provide adequate symptom relief without a protracted treatment course is undoubtedly a valuable tool.
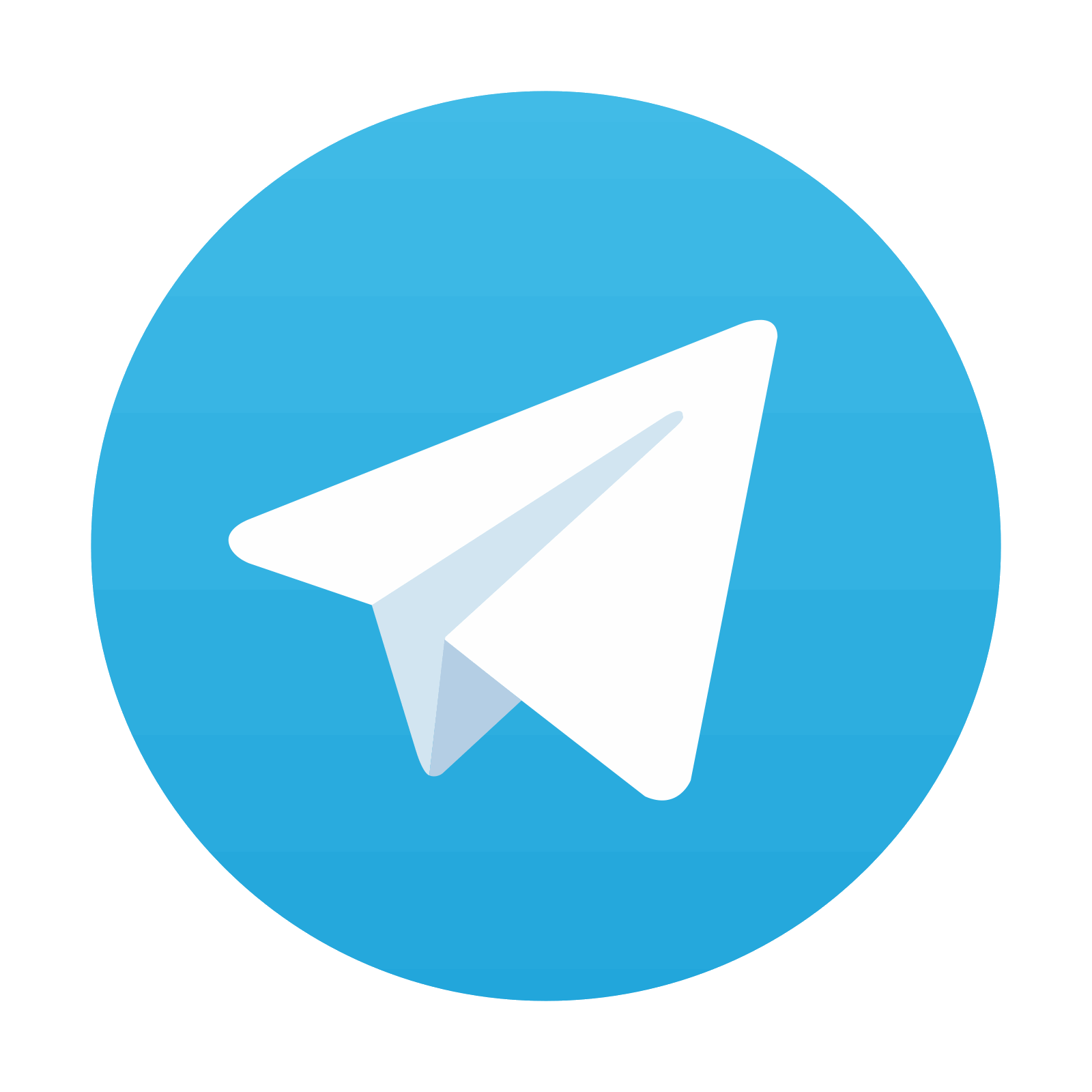
Stay updated, free articles. Join our Telegram channel
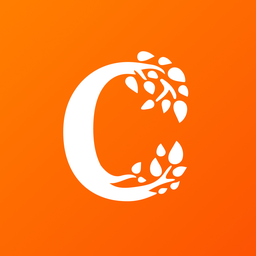
Full access? Get Clinical Tree
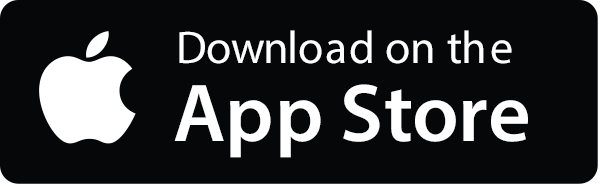
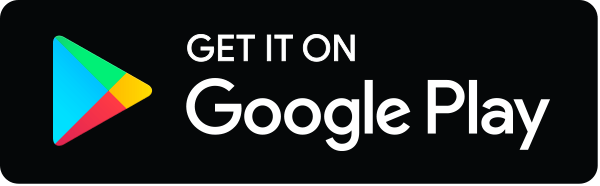