Fig. 1.1
The power of proteomics. A proteomic comparison of tryptic peptides isolated from the spermatozoa of patients whose gametes lack a capacity to bind to the zona pellucida demonstrated the central importance of a heat shock protein, HSPA2, in orchestrating this physiological process [76–78]. (a) Extracted ion chromatogram demonstrating the difference in expression of a specific peptide which was traced to the HSP70 family. (b) MS/MS spectrum of the peptide. (c) A small window from the MS survey scan demonstrating the difference in peptide expression between the patient’s spermatozoa and a normal fertile control
While such work highlights the utility of comparative proteomic strategies as a powerful starting point for dissecting the functional lesions associated with male infertility, the main disadvantages of this approach are concerned with post-experimental data processing. Indeed, the extremely large volume of data collected in such experiments presents a significant problem in terms of both the time required to collate and assemble the data into a useable format and the computing power needed to complete database searching. This problem may eventually be alleviated as mass spectrometric instrumentation, sequencing algorithms, and the performance of computing resources continue to improve and become more affordable. These improvements, coupled with the increasing stringency of data requirements of journals are helping to make results more transparent and address the burden of erroneous identification of proteins that appear in many published proteomes [90].
SRM/SWATH-MS
Researchers in the field of human sperm biology have traditionally been quick to embrace innovative developments in proteomic analyses. However, to the best of our knowledge there are presently few reports capitalizing on recent technological breakthroughs in label-free quantitative proteomics, such as Selected Reaction Monitoring [(SRM), also known as Multiple Reaction Monitoring (MRM)], Parallel Reaction Monitoring (PRM), and Sequential Windowed Acquisition of all Theoretical fragment ion spectra-mass spectrometry (SWATH-MS) analyses. Driven by recent advances in the speed and sensitivity of the new generation of high resolution mass spectrometry instrumentation, these technologies afford the ability to not only determine which proteins are present in the sperm proteome, but also to accurately quantitate them in a variety of biological contexts at a resolution that far exceeds that obtained using traditional quantitative approaches. As such, these techniques are being heralded as among the most important recent developments in proteomics research.
SRM is an absolute quantitation method that exploits the unique capabilities of a triple quadrupole mass spectrometer. This analysis is performed by the acquisition of selected events across the LC retention time domain of predefined pairs of precursor and product ion masses. The technique becomes an absolute quantitation tool by spiking isotope-labeled synthetic peptide(s) into the complex sample of interest, which acts as an internal standard for any peptide(s) of interest. The labeled peptide standards are designed to mimic those generated by tryptic sample digestion, thus enabling them to co-elute and be subjected to MS/MS analysis along with the target peptides. A calibration-response curve based on the labeled peptides is subsequently used to accurately determine the absolute concentration of targeted peptides, a procedure that is repeated for each target within the sample. It follows that assay development and optimization are key elements of the SRM proteomic strategy. Indeed, the labeled peptides must be synthesized based on a priori knowledge for each target, taking into account those tryptic fragments that possess optimal electrochemical characteristics. While sophisticated software packages are available to help predict the most suitable peptide sequences, an element of trial-and-error and the necessity for instrument optimization renders the process of quantitation via SRM a time-consuming and costly endeavor. Notwithstanding such limitations, SRM is now firmly established as a method of choice for quantitative clinical applications. This reflects its unparalleled ability to characterize and quantify a set of proteins reproducibly, selectivity and with high sensitivity. Indeed, SRM readily extends analytical capability to low-abundance proteins without bias from abundant analytes, with recent reports suggesting the technique can detect proteins with as few as 50 copies per cell from among complex unfractionated lysates [91].
In one of the first applications to illustrate the potential of SRM in the context of male infertility research, Drabovich et al. employed the technique to assess a cohort of prospective seminal plasma biomarkers for their ability to discriminate between fertile, post-vasectomy and non-obstructive azoospermia patients [92]. From an initial investigation of 31 proteins identified in a multiplex SRM assay, the authors synthesized heavy isotope-labeled internal standards to reanalyze the concentration of 20 of the most promising candidates [92]. This approach was subsequently extended through investigation of diagnostic biomarkers to differentiate between obstructive and non-obstructive azoospermia [93]. In both instances, key biomarkers were identified that performed with either absolute, or nearly absolute, specificities and sensitivities in these assays. Such results offer the promise of developing viable alternatives to alleviate the current need for invasive testicular biopsy as the only definitive diagnostic method to distinguish between obstructive and non-obstructive azoospermia.
The recent advent of faster acquisition MS equipment has fueled the development of a new proteomic approach referred to as SWATH-MS. In essence, SWATH-MS allows the generation of a complete and permanent spectral library constituting a record of all fragment ions of the peptide precursors present in a biological sample. In combining the unique and material advantages of traditional shotgun (high throughput) and SRM (high reproducibility and consistency) technologies, SWATH-MS can be deployed for both discovery and quantitation of all detectable peptides present in complex biological samples. It also affords the added advantage that it does not rely on prior knowledge of the precursor peptide ions, instead acquiring information in a data-independent manner and thus avoiding laborious assay development. The SWATH-MS workflow involves two key steps beginning with the generation of a spectral library (e.g. via conventional LC-MS/MS) through which acquired peptides are identified. During this acquisition mode, the mass spectrometer is programed to step within 2–4 s cycles through a set of precursor acquisition windows covering the mass range accessible by a quadrupole mass analyzer and also that in which most tryptic peptide precursors should fall (400–1200 m/z). During each cycle, the mass spectrometer fragments peptide precursors and records a complete, high accuracy fragment ion spectrum for all precursors that elute on the chromatograph. This is then followed by acquisition of SWATH-MS data for each sample under analysis, interrogating and matching against the spectral library to identify peptides, and finally extraction of specific peptide ions to enable area-under-the-curve quantitation between samples.
1.3 Sub-cellular/Proteomic Fractionation Strategies
Notwithstanding the significant advances this next generation of proteomic technologies is likely to afford in terms of defining the complete human sperm proteome, a considerable challenge that lies ahead rests with our ability to convert such a vast body of data into meaningful biological function [21]. In an effort to realize the transformative potential of this resource, there is an increasing interest in coupling comparative proteomics with methods of subcellular fractionation and protein/peptide enrichment techniques for investigation of the key functional domains and post-translational modifications that are required to achieve successful fertilization.
In this context, several groups have begun to characterize important sub-proteomes associated with human sperm capacitation. A focus for these studies has been analysis of the phosphoproteome of capacitated human spermatozoa utilizing pre-fractionation strategies in which phosphopeptide enrichment is coupled with MS/MS [94] or label-free quantitative phosphoproteomics [81]. In the former study, more than 60 phosphorylated sequences were mapped leading to the identification of novel targets for capacitation-associated tyrosine phosphorylation including: valosin-containing protein, a homolog of the SNARE-interacting protein NSF, and A-kinase anchoring protein types 3 and 4 [94]. In the latter study, an expanded cohort of some 3,303 phosphorylation sites, corresponding to 986 proteins, were identified following immobilized metal affinity chromatography (IMAC)-TiO2 phosphopeptide enrichment. Among these candidates, the phosphorylation levels of 231 sites were increased significantly, including that of insulin growth factor 1 receptor, a tyrosine receptor kinase implicated in the regulation of hyperactivated motility [81]. Such studies have also recently been extended to provide valuable insight into the functional impact of alternative forms of post-translational modification including: S-nitrosylation [95], lysine acetylation [96, 97], lipid aldehyde (4-hydroxynonenal) adduction [98], N-glycosylation [99], and sumoylation [100] on human spermatozoa.
Among the common themes emerging from these studies is the striking increase in proteome complexity generated by the dynamic post-translational modification that accompany sperm development and post-testicular maturation processes. Indeed, with in excess of 400 different forms of post-translational modification, it is likely that we are only beginning to scratch the surface of the crucial role such modifications play in sperm physiology and pathology. This notion is reinforced by the relatively poor correspondence between the capacitation-associated phosphoproteomes identified in the spermatozoa of humans as opposed to those of model species such as the mouse [101], rat [102], and hamster [103]. While it is difficult to refute the contribution of methodological differences, these data also speak to the possibility that a large portion of the phosphoproteome remains unexamined.
One of the most promising approaches to reduce this overall complexity is the use of subcellular fractionation strategies that seek to break the cell down into its constituent parts prior to MS analysis. Indeed, as one of the most highly differentiated cell types in the human body, spermatozoa are uniquely amenable to this form of analysis. Accordingly, several studies have begun to emerge in which the protein signature of discrete functional domains has been reported. These proteomic catalogues now include membrane microdomains involved in mediation of oocyte interactions (plasma membranes [47, 104, 105], detergent resistant membranes [106]), the sperm nucleus [107], chromatin [108, 109], head [110], and flagellum [110–112]). Through the reduction of the dynamic range and enrichment of less abundant proteins, these combined approaches have helped increase our coverage of the human sperm proteome and apportion protein function to specific subcellular domains [110]. A central tenet of this work has been the marked division of labor that exists among sperm proteins/domains. Thus, among a total of 1,429 proteins successfully identified in their comparative proteomic analysis of the human sperm head and flagellum, Baker et al. [110] reported only 179 (~12 %) proteins that were detected in both cellular domains. In addition to the anticipated partitioning of metabolic enzymes within the flagellum, the sperm head featured an abundance of proteasomal and signaling machinery. These findings mirror those reported in a more comprehensive analysis of the sperm flagellum proteome in which Amaral et al. identified a total of 1,049 proteins [111]. Interestingly, however, less than half of these flagellum proteins were identified in both datasets [110, 111], illustrating that we still have some considerable ground to cover before achieving the ultimate goal of documenting the complete sperm proteome.
Conclusions
Simultaneous advances in mass spectrometry design, computing power, and the availability of genomic sequence data for a variety of organisms have fueled rapid growth in the field of proteomic analysis and served to enhance the utility of this approach for the study of human sperm function. Ambitious, large-scale, mass-spectrometry-based proteomic analyses have identified complex inventories comprising thousands of sperm proteins with a dynamic range of abundance of several orders of magnitude. In fact, obtaining mass-spectrometry data has already ceased to be the limiting step in sperm proteomics. Instead, the main challenge that lies ahead is to exploit this valuable resource in order to define which specific elements of the proteome are of functional significance and understand the cascade of post-translational modifications involved in generating a functional spermatozoon. Ultimately the success of such studies will be measured by our progress in understanding the molecular mechanisms that may be targeted for contraceptive purposes or implicated in the etiology of defective sperm function.
References
1.
Hermo L, Pelletier RM, Cyr DG, Smith CE (2010) Surfing the wave, cycle, life history, and genes/proteins expressed by testicular germ cells. Part 1: background to spermatogenesis, spermatogonia, and spermatocytes. Microsc Res Tech 73:241–278PubMed
2.
Gadella BM, Lopes-Cardozo M, van Golde LM, Colenbrander B, Gadella TWJ (1995) Glycolipid migration from the apical to the equatorial subdomains of the sperm head plasma membrane precedes the acrosome reaction. Evidence for a primary capacitation event in boar spermatozoa. J Cell Sci 108:935–946PubMed
3.
Phelps BM, Primakoff PK, Koppel DE, Low MG, Myles DG (1988) Restricted lateral diffusion of PH-20, a PI-anchored sperm membrane protein. Science 240:1780–1782PubMed
4.
Baker MA, Nixon B, Naumovski N, Aitken RJ (2012) Proteomic insights into the maturation and capacitation of mammalian spermatozoa. Syst Biol Reprod Med 58:211–217PubMed
5.
Anway MD, Li Y, Ravindranath N, Dym M, Griswold MD (2003) Expression of testicular germ cell genes identified by differential display analysis. J Androl 24:173–184PubMed
6.
Catalano RD, Vlad M, Kennedy RC (1997) Differential display to identify and isolate novel genes expressed during spermatogenesis. Mol Hum Reprod 3:215–221PubMed
7.
O’Shaughnessy PJ, Fleming L, Baker PJ, Jackson G, Johnston H (2003) Identification of developmentally regulated genes in the somatic cells of the mouse testis using serial analysis of gene expression. Biol Reprod 69:797–808PubMed
8.
Aguilar-Mahecha A, Hales BF, Robaire B (2001) Expression of stress response genes in germ cells during spermatogenesis. Biol Reprod 65:119–127PubMed
9.
Almstrup K, Nielsen JE, Hansen MA, Tanaka M, Skakkebaek NE, Leffers H (2004) Analysis of cell-type-specific gene expression during mouse spermatogenesis. Biol Reprod 70:1751–1761PubMed
10.
Guo R, Yu Z, Guan J, Ge Y, Ma J, Li S, Wang S, Xue S, Han D (2004) Stage-specific and tissue-specific expression characteristics of differentially expressed genes during mouse spermatogenesis. Mol Reprod Dev 67:264–272PubMed
11.
Yu Z, Guo R, Ge Y, Ma J, Guan J, Li S, Sun X, Xue S, Han D (2003) Gene expression profiles in different stages of mouse spermatogenic cells during spermatogenesis. Biol Reprod 69:37–47PubMed
12.
Yang Q, Hua J, Wang L, Xu B, Zhang H, Ye N, Zhang Z, Yu D, Cooke HJ, Zhang Y, Shi Q (2013) MicroRNA and piRNA profiles in normal human testis detected by next generation sequencing. PLoS One 8:e66809PubMedPubMedCentral
13.
Aston KI, Carrell DT (2009) Genome-wide study of single-nucleotide polymorphisms associated with azoospermia and severe oligozoospermia. J Androl 30:711–725PubMed
14.
Zhang Y, Li Q, Wu F, Zhou R, Qi Y, Su N, Chen L, Xu S, Jiang T, Zhang C, Cheng G, Chen X et al (2015) Tissue-based proteogenomics reveals that human testis endows plentiful missing proteins. J Proteome Res 14:3583–3594PubMed
15.
Carrell DT, Aston KI, Oliva R, Emery BR, De Jonge CJ (2016) The “omics” of human male infertility: integrating big data in a systems biology approach. Cell Tissue Res 363:295–312PubMed
16.
Wang G, Guo Y, Zhou T, Shi X, Yu J, Yang Y, Wu Y, Wang J, Liu M, Chen X, Tu W, Zeng Y et al (2013) In-depth proteomic analysis of the human sperm reveals complex protein compositions. J Proteomics 79:114–122PubMed
17.
Codina M, Estanyol JM, Fidalgo MJ, Ballesca JL, Oliva R (2015) Advances in sperm proteomics: best-practise methodology and clinical potential. Expert Rev Proteomics 12:255–277PubMed
18.
Oliva R, De Mateo S, Castillo J, Azpiazu R, Oriola J, Ballesca JL (2010) Methodological advances in sperm proteomics. Hum Fertil (Camb) 13:263–267
19.
Amaral A, Castillo J, Ramalho-Santos J, Oliva R (2014) The combined human sperm proteome: cellular pathways and implications for basic and clinical science. Hum Reprod Update 20:40–62PubMed
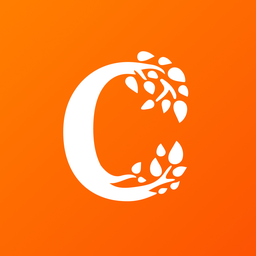
Full access? Get Clinical Tree
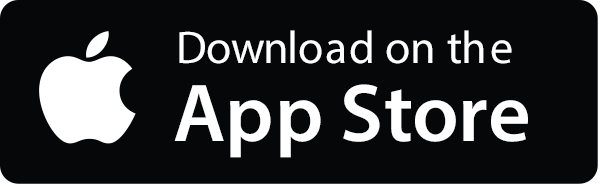
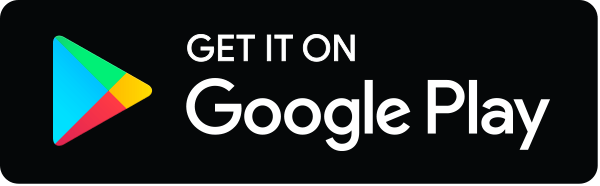