CHAPTER 36 Principles of radiotherapy and chemotherapy
Radiotherapy
Radiotherapy is the utilization of ionizing radiation, primarily for the treatment of malignant cancers and infrequently for some preinvasive and benign conditions. It is central to the curative treatment of women with cervical carcinoma, and is used in the adjuvant and palliative treatment of other gynaecological malignancies.
Radiation physics
Therapeutic ionizing radiation can be electromagnetic or particulate. Particulate radiation causes ionization directly and electromagnetic radiation indirectly by ejecting fast-moving electrons from atoms, resulting in potentially significant biochemical, cellular and tissue changes within biological systems. Radiation energy absorbed in tissue is measured in grays (Gy), with 1 Gy being equivalent to 1 joule per kilogram.
Electromagnetic radiation
X-rays and gamma-rays (γ-rays) are part of the electromagnetic spectrum; the former are produced artificially and the latter from naturally occurring or artificially produced radioisotopes. Megavoltage X-rays for therapeutic purposes are produced by a linear accelerator (Figure 36.1) by accelerating electrons to high kinetic energies on to a target composed of tungsten or gold. Kinetic energy given up by the electrons is converted to high-energy X-rays. γ-rays are emitted as byproducts of radioactive isotopes (e.g. iridium-192) undergoing decay to reach a stable nuclide.
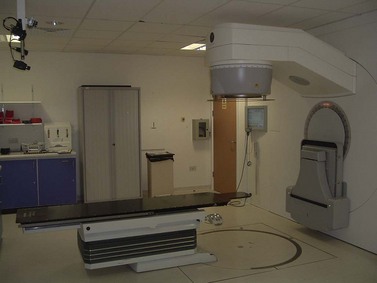
Figure 36.1 A linear accelerator and radiotherapy treatment room. The linear accelerator can rotate 360° around the treatment couch, which is also able to rotate about its axis, allowing the patient to be treated using beams from multiple directions. The patient is monitored during treatment using cameras, and communication is possible using a microphone.
X-rays and γ-rays are characterized by very short wavelengths and high frequencies, and consist of packets of energies (photons) capable of tissue ionization. Higher energy photons have greater tissue penetration. Photon energies in the range of 6–15 million electron volts (megavolts, MV) are typically used for pelvic irradiation, although higher energies may be required for obese patients to achieve adequate dose to the centre of the pelvis. Use of photon energies less than 4 MV can result in greater dose variation within the patient.
Particulate radiation
This consists of atomic subparticles: electrons (negatively charged), protons (positively charged), neutrons (no charge) and negative π-mesons. Only electrons are commonly used in clinical practice. High-energy electrons are produced in a linear accelerator from which the target has been removed. Electrons react more rapidly with tissue compared with photons, and have a depth–dose profile which follows a plateau for a distance depending on the electron energy, followed by a rapid dose fall-off. Electrons are useful for the treatment of superficial tumours, allowing sparing of deeper structures which reduces treatment-related morbidity.
Proton beam radiotherapy has generated increasing interest in recent years. The dose distribution of a monoenergetic proton beam increases slowly with depth but reaches a sharp maximum near the end of the particle’s range (Bragg peak) with subsequent rapid dose fall-off. This has the attraction of precisely confining the high-dose region to the deep-seated tumour volume whilst minimizing the dose to the surrounding normal tissues. The availability of proton beam therapy remains restricted by the limited number of cyclotron machines available globally for the production of protons.
Radiobiology
Radiobiology is the study of the effects of ionizing radiation on biological systems. Ionizing radiation acts on both normal and cancer cells. The primary ionization event in living cells usually occurs within intracellular water molecules, with subsequent ionization occurring in surrounding macromolecules. Deoxyribose nucleic acid (DNA) is the critical intracellular target for radiation therapy. Following DNA damage, some cells undergo immediate apoptosis. More frequently, death occurs at subsequent mitosis and the main effect of radiation is to delay or prevent mitosis. The onset of the latter depends on the cellular turnover time, hence more rapidly dividing systems (e.g. intestinal epithelium) show the effects of radiation earlier compared with slowly dividing systems (e.g. central nervous system).
Eradication of a cancer is only possible if irreversible DNA damage in all tumour clonogens is achieved. The success or otherwise of ionizing radiation in achieving this is governed by the five ‘Rs’ of radiobiology: reoxygenation, redistribution, repair, repopulation and (intrinsic) radiosensitivity. Intracellular oxygen is important for the ionizing effects of radiation, and total hypoxia results in a dose reduction of a factor of 2–3 in most biological systems. Reoxygenation of hypoxic cells occurs as a consequence of tumour regression during fractionated radiotherapy, resulting in increased radiation efficacy. Anaemia is a poor prognostic factor in the treatment of cervical carcinoma, and is thought to relate to reduced oxygen partial pressures secondary to a low haemoglobin concentration. Patients having radiotherapy for cervical carcinoma should have their haemoglobin concentration maintained above 12 g/dl. Redistribution of cells into the cell cycle from the resting phase during a course of fractionated radiotherapy increases the number of cells undergoing mitotic division, increasing the probability of tumour cell kill. Despite radiation, some tumour clonogens may undergo successful repair of DNA damage, and subsequent repopulation of these cells results in treatment failure. Finally, intrinsic radiosensitivity is an inherent property of tumours; some tumours (e.g. lymphomas) are successfully eradicated with relatively low doses of radiotherapy, whilst others (e.g. gliomas) frequently recur despite high radiation doses. Indirect effects of radiation on tumours include loss of small blood vessels leading to tumour necrosis and subsequent activation of the reticuloendothelial system.
A major challenge for radiotherapists is to eradicate tumours without causing significant permanent damage to normal tissue. Three important factors need consideration when planning a radical course of radiation: the total dose of radiation required to achieve tumour eradication, the number of fractions required to deliver the total dose, and the length of time over which the treatment is delivered. Normal tissues have a greater capacity for repair of sublethal DNA damage compared with cancer cells, provided that the overall dose is not too large and is given over a period of time. However, significant prolongation of treatment increases the risk of treatment failure for some cancers, including squamous cell carcinoma of the cervix. Advantages of fractionated radiotherapy include:
Normal tissue tolerance is an important concept in radiotherapy. Accepted tolerance ranges of total radiation dose which result in a clinically acceptable low incidence of treatment-related morbidity have been established. A number of factors adversely influence the radiation tolerance of normal tissues, including: volume of tissue irradiated, previous surgery, exposure to previous or concomitant cytotoxic treatment, vascular insufficiency, diabetes mellitus and connective tissue disorders.
Concomitant chemoradiation
Cisplatin-based chemoradiation became the standard of care in cervical cancer following an alert issued by the National Cancer Institute in 1999. The benefit of chemoradiation in cervical cancer over radiotherapy alone has been confirmed by recent meta-analyses. Concomitant chemotherapy may have an additive or synergistic effect when combined with radiotherapy. Perceived benefits include increased tumour sensitization to radiotherapy and eradication of systemic micrometastatic disease.
Radiation dose and scheduling
Radiotherapy fractionation
Conventional practice involves once-daily treatment giving five fractions per week using 1.8–2 Gy/fraction. Modified dose-fractionation schedules have been investigated in an attempt to improve cure rates. Hyperfractionated radiotherapy is the delivery of two or more fractions per day using smaller doses per fraction to deliver a higher total dose over the same time period compared with standard fractionated radiotherapy. An interfraction gap of a minimum of 6 h is recommended for recovery of normal cells. Advantages of this approach include delivery of a higher total dose to improve tumour eradication whilst allowing sufficient time for normal tissue repair between fractions. Accelerated radiotherapy refers to the delivery of radiotherapy over a significantly shorter period of time by delivering six or more fractions per week, with an interfraction gap of a minimum of 6 h. The total dose and dose per fraction remain the same as for standard treatment. Delivering radiotherapy over a shorter period of time reduces the negative effects of accelerated repopulation seen in some cancers. Hypofractionated radiotherapy delivers radiation treatment over a shorter period of time by using fraction sizes larger than 2 Gy/fraction. However, such an approach increases the risk of late normal tissue toxicity, as normal tissues are more sensitive to larger doses per fraction compared with tumour cells. In order to reduce this risk, the total dose of radiotherapy is reduced to deliver the same biologically effective dose. Hypofractionated radiotherapy is commonly used in palliative radiotherapy to minimize patient inconvenience and to optimize resource utilization.
Dose prescription
A radiotherapy prescription specifies the total dose to be given, the total number of fractions to be used, the dose per fraction, and the overall treatment duration. The total dose required to eradicate a tumour is dependent on the tumour volume to be treated. Whereas a dose of 50 Gy in 25 fractions treating five times per week over 5 weeks is sufficient to eradicate microscopic disease in 90% of cases, doses above 66 Gy using 2 Gy/fraction over 6.5 weeks are required to eradicate macroscopic disease. Where delivery of radical radiotherapy with curative intent is not possible because of tumour-related (e.g. tumour volume, metastatic disease) or patient-related (e.g. poor performance status) factors, palliative radiotherapy may be considered to alleviate distressing symptoms. Doses typically used include 8 Gy in a single fraction, 20 Gy in five fractions over 1 week or 30 Gy in 10 fractions over 2 weeks.
Radiotherapy techniques
Teletherapy, also referred to as ‘external beam radiotherapy’, and brachytherapy are commonly utilized in the treatment of gynaecological malignancies and are discussed below. Instillation of radioactive fluids into the peritoneal or pleural cavity for treating small volume disease is rarely used and will not be discussed in detail.
External beam radiotherapy
This is irradiation of tumours at a ‘long’ distance away from the source of ionizing radiation. Pelvic external beam radiotherapy remains integral to the treatment of gynaecological malignancies as it permits irradiation of the primary tumour and regional lymph nodes. The dose that can be delivered to the tumour is limited by the tolerance of the normal tissues, also referred to as the ‘organs at risk’, which include the small bowel, rectum and bladder. Technological advances in the methods of radiation delivery are increasingly permitting the use of techniques which minimize the dose received by normal tissues whilst allowing a higher dose to be delivered to the tumour. Radiotherapy treatment planning remains a vital component of radiation treatment.
Radiotherapy planning
The aim of radiotherapy planning is to deliver a homogenous dose to the primary tumour and potential areas of micrometastatic disease whilst minimizing the dose to the organs at risk. Accurate tumour localization is central to radiotherapy planning, and it is important to have the following information to hand: findings of clinical examination including examination under anaesthesia, operation notes and intraoperative findings, histopathology report and the results of any radiological investigations including computed tomography (CT), magnetic resonance imaging (MRI) and 18FDG-positron emission tomography (PET) scans.
Simulator-based radiotherapy planning has been superseded by CT-based planning which allows more accurate visualization of the tumour and organs at risk. Where appropriate, coregistration of MRI and CT images provides greater certainty and clarification of anatomy. Coregistration of functional imaging scans (e.g. PET scan) with planning CT scans may provide yet more sophisticated radiotherapy planning. The simulator — a diagnostic X-ray machine connected to a television screen which emulates a treatment machine — continues to have a useful role for palliative radiotherapy planning. It is also useful in the minority of cases where CT scanning is not possible due to patient obesity or other patient-related factors.
Planning procedure
A vaginal examination is performed prior to the CT scan to determine the extent of vaginal involvement and the most inferior aspect of the tumour. The patient is scanned in the supine position unless a belly board is used to displace the small bowel anteriorly to minimize small bowel irradiation, in which case she is placed in the prone position. A vaginal probe can aid in defining the extent of vaginal involvement, although this is not universally utilized. Rectal contrast may be used to delineate the rectum and sigmoid. Intravenous contrast may be administered to enhance identification of blood vessels which are anatomical landmarks for the regional lymph nodes. A CT scan is performed using 2.5–5-mm slices from the third lumbar vertebra to 5 cm below the ischial tuberosities. The superior border is extended to include the lower thoracic vertebrae if para-aortic lymph node irradiation is required. The patient is asked to have a comfortably full bladder to reduce the volume of small bowel within the pelvis. Following acquisition of CT images, permanent tattoos are marked on the patient’s skin as reference points for aligning her in the correct position for each fraction of treatment.
Conformal radiotherapy
The CT scan images are subsequently transferred to a computer terminal (Figure 36.2) with software allowing delineation of target volumes and organs at risk according to recommendations from the International Commission on Radiation Units and Measurements. The gross tumour volume includes the palpable and/or radiologically visible tumour; it is absent if it has been surgically resected. The clinical target volume (CTV) involves the gross tumour volume and potential areas of microscopic disease (e.g. pelvic lymph nodes and parametria in cervical cancer). The CTV is grown by a margin to allow for internal organ motion and geometric uncertainties in treatment delivery; this is called the ‘planning target volume’ (PTV). The organs at risk for pelvic radiation include the rectum, bladder, small bowel and both hip joints. The kidneys and spinal cord are the dose-limiting organs at risk when radiating the para-aortic lymph nodes. The clinician specifies the dose fractionation to be used in the radiation treatment of the patient.
Following the process described above, a radiotherapy treatment plan is produced by medical physicists using complex computer algorithms which simulate the effects of a radiation beam passing through the designated area and the radiation dose deposited at any one site. Three or more beams are typically required to produce a homogenous dose for the radiation of pelvic cancers; the use of two beams increases the volume of bowel receiving radiotherapy and risk of radiation enteropathy. However, the use of two opposing anterior–posterior beams is necessary for irradiation of carcinoma of the vulva due to irradiation of inguinal lymph nodes. Modern linear accelerators contain computer-driven multi-leaf collimators which shape the radiation beams to improve conformity to the PTV and to shield the organs at risk. These have replaced the lead blocks previously manually placed in the beam to provide shielding of the organs at risk, thus speeding up the treatment process and reducing the heavy manual work required of therapy radiographers.
The final radiotherapy plan comprises the following information: the number of beams used to optimize conformity to the PTV, beam energies, beam direction, use of wedge angles and multi-leaf collimators to improve PTV conformity, and the dose distribution and percentage dose received by the PTV. A dose–volume histogram is provided to determine the dose being received by the PTV and organs at risk. The final plan is checked and approved by the clinician prior to implementation of the radiotherapy plan (Figure 36.3). Verification checks are performed to validate the treatment plan and to detect for any unforeseen errors prior to starting radiation treatment. A standard dose prescription for carcinoma of the cervix is 45–50 Gy in 25–28 fractions using 1.8 Gy/fraction over 5–5.5 weeks.
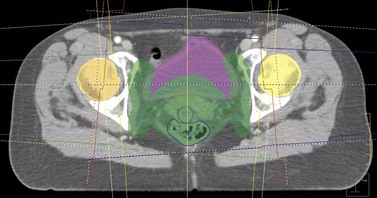
Figure 36.3 A computed tomography (CT) slice from a four-field conformal radiotherapy plan to treat a patient with carcinoma of the cervix. The green area represents the clinical target volume (CTV) consisting of the primary tumour plus a margin for microscopic disease and the pelvic nodes. The organs at risk are also drawn on the CT slice: rectum (blue), urinary bladder (purple) and femoral heads (yellow).
Image courtesy of Professor Peter Hoskin, Mount Vernon Hospital, Northwood.
The patient is treated in a specially designed room to prevent radiation of personnel outside the room. The patient is positioned on the treatment couch with reference to the tattoo marks (Figure 36.4). Computerized transfer of planning data from the planning computer to the linear accelerator minimizes the risk of human error in transferring data. The radiographers control the linear accelerator from outside the room using a console which is used to start and stop the radiation beam, and to set the duration of treatment and the dose to be delivered. The patient is monitored using cameras in the treatment room, and communication with the patient is possible via a microphone. These safety features are designed to optimize radiation treatment and are subject to frequent quality assurance checks.
Virtual simulation
The planning CT scan images can be reconstructed using computer software to provide a digitally reconstructed radiograph (DRR). Bony landmarks are used for radiotherapy planning with the clinician using the computer software to place the radiation beams on to the DRR. This is akin to conventional radiotherapy. The advantages of virtually simulated radiotherapy planning include: the clinician is able to visualize the pelvic soft tissues by using the CT scan images, thus ensuring the tumour is encompassed within the radiation field; radiotherapy planning is less time-consuming compared with conformal radiotherapy; the clinician can apply multi-leaf collimators at the time of planning to optimize shielding of the organs at risk; and a dose distribution can be provided by the medical physicists to ensure that the PTV is receiving the prescribed dose. The disadvantages of virtually simulated radiotherapy planning are that it is not suitable for producing complex radiotherapy plans, and a dose–volume histogram cannot be provided to determine the dose being received by the organs at risk. Virtually simulated radiotherapy (Figure 36.5) is frequently utilized for pelvic radiotherapy for the reasons outlined above.
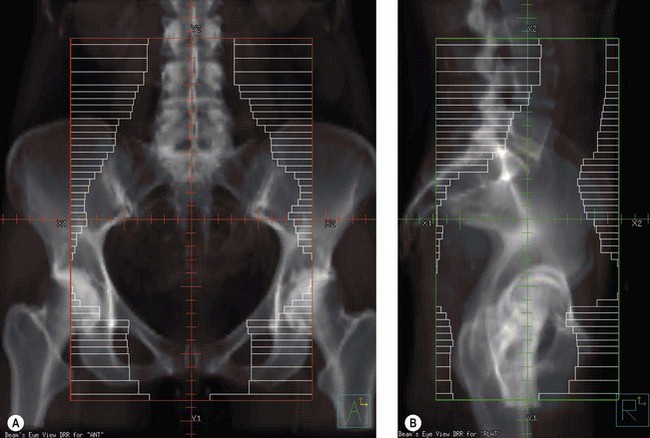
Figure 36.5 Anterior (A) and right lateral (B) views of a virtually simulated radiotherapy plan for carcinoma of the cervix. The superior border has been extended superiorly to include the common iliac lymph nodes. The red (A) and green (B) delineated areas denote the planning target volume; the white rectangles represent the multi-leaf collimators used to shield organs at risk which do not need to be irradiated.
Images courtesy of Professor Peter Hoskin, Mount Vernon Hospital, Northwood.
Conventional radiotherapy
Conventional external beam radiotherapy uses bony landmarks to define the target volume for pelvic radiotherapy. A simulator film is obtained after the patient is aligned on the simulator couch using orthogonal lasers. Cross wires mounted in the light beam from the simulator define the size of the area to be treated, and anterior–posterior and lateral pelvic X-rays are obtained as permanent records. The clinician is able to identify areas where shielding can be applied to reduce dose to the organs at risk (Figure 36.6). As diagnostic X-rays have poor soft tissue resolution, the clinician must rely on information gathered from clinical examination and radiological investigations to determine the target volume. Studies have reported underdosing of the regional lymph nodes in up to 40% of patients using conventional radiotherapy. However, conventional radiotherapy planning still has a role in centres where access to CT planning software is restricted due to resource implications, in patients unable to fit into a CT scan machine due to gross obesity, and in palliative radiotherapy.
Intensity-modulated radiotherapy
Intensity-modulated radiotherapy (IMRT) is generally regarded as a significant advancement in the development of conformal radiotherapy. A major advantage of IMRT is the ability to generate PTVs which conform to targets which are concave in the plane of the incident beams, which is not possible with standard conformal radiation. This is achieved by using numerous non-coplanar beams of varying intensity to improve conformity to treatment targets whilst further reducing the dose to selected organs at risk. However, IMRT results in a larger volume of normal tissue receiving low doses of radiation; one of the concerns of IMRT is that, over a period of time, this may result in a higher incidence of secondary malignancies and other unwanted late toxicities.
IMRT may be inverse- or forward-planned. In inverse-planned IMRT, the clinician specifies the dose constraints within the patient and an inverse-planning algorithm computes the beam modulations required to produce the specified dose distribution. A number of planning cycles may be required to produce an ‘optimized’ dose distribution. Forward-planned IMRT utilizes a limited number of non-coplanar beams of different weighting to deliver a more conformal plan. An additional advantage of IMRT is that it is possible to allocate different dose targets within a single treatment volume by a process called ‘simultaneous integrated boost IMRT’.
A number of studies investigating the role of IMRT in cervical and endometrial cancer have been published in the literature. The majority are single-centre retrospective analyses involving fewer than 100 patients.
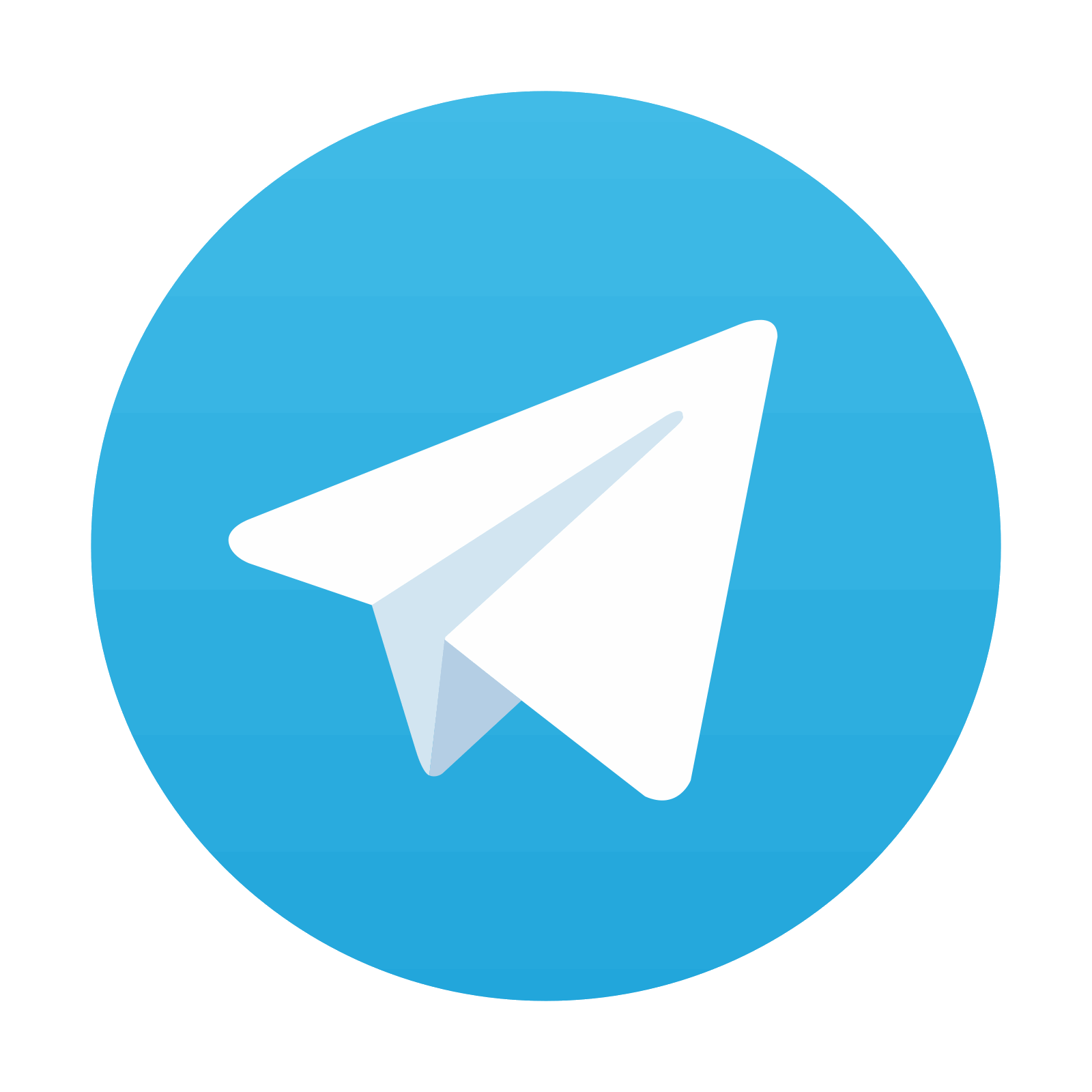
Stay updated, free articles. Join our Telegram channel
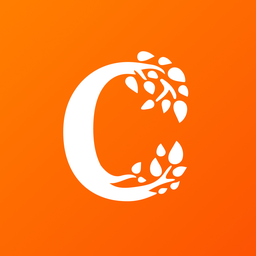
Full access? Get Clinical Tree
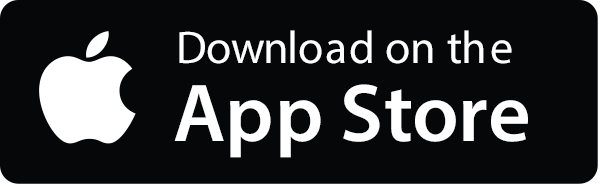
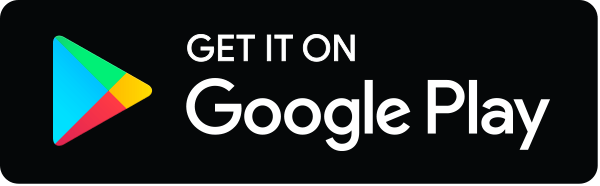