Principles of Chemotherapy in Gynecologic Cancer
HISTORICAL OVERVIEW OF CANCER CHEMOTHERAPY
We are in the midst of an important transition from conventional cytotoxic agents to new strategies that incorporate molecular-targeted therapeutics. Even with these changes in our treatment paradigm, several conventional cytotoxic agents maintain a central role in the care of women with gynecologic cancers. In that context, a brief review of drug development provides a framework to understand the evolving balance between toxicity, efficacy, and design of treatment regimens.
The first report of a drug-mediated tumor response was noted over 125 years ago using Fowler’s solution (arsenic trioxide in potassium bicarbonate) in patients with Hodgkin’s disease and leukemia (1). Arsenic compounds had been used for various medicinal purposes for over 2,000 years, and it was not surprising that they were tested in patients with cancer. Cyclic hematologic toxicity was observed following arsenic administration in normal individuals and patients with leukemia (2), establishing a close association between tumor response and host toxicity that still exists today. Cumulative dose-limiting toxicity (arsenic poisoning) was also described following expanded utilization of arsenic in chronic myelogenous leukemia (3), coinciding with a transition toward radiation therapy for the management of leukemia and lymphoma around 1940.
The term chemotherapy has been attributed to Paul Ehrlich, a Nobel laureate physician and bacteriologist, who developed in vivo rodent models of infection, including the introduction in 1910 of Salvarsan, an organic arsenical originally used to cure syphilis and still used in the management of trypanosomiasis. His early in vivo modeling also encouraged the development of inbred transplantable rodent tumors, thereby establishing a paradigm that has been widely adopted for screening new antitumor agents.
Although the topical vesicant properties of sulfur mustard received much attention during World War I, multiple systemic effects, including leukopenia, bone marrow aplasia, and mucosal ulceration, emerged with further study and would ultimately have greater importance. Cancer chemotherapy, in the traditional sense, began with the demonstration that nitrogen mustard had reproducible activity against transplanted lymphoma in mice, prompting clinical trials as early as 1942 (4). However, owing to World War II, much of the research remained classified until 1946. Following the demonstration by Farber in 1948 that aminopterin, an antifolate, could induce temporary remission in childhood leukemia (5), antimetabolites became the next major category of agents to be developed, and were ultimately associated with cures in women with choriocarcinoma (6). Research during the 1940s also included the Nobel Prize–winning observations of Huggins and others (7) regarding the antitumor effect of estrogens in prostatic cancer.
Conventional Cytotoxic Agents
Between 1945 and 1965, many important chemotherapeutic agents, such as actinomycin D, cyclophosphamide, 5-fluorouracil, Vinca alkaloids, and progestogens, were developed and demonstrated to have antitumor activity in clinical trials. Between 1965 and 1975, the pace of new drug discovery and development continued. During this period, cisplatin was shown to have activity in testicular and ovarian tumors, and doxorubicin, bleomycin, etoposide, and tamoxifen entered our clinical arsenal. This was followed by the identification of analogs, such as carboplatin, idarubicin, and vinorelbine, with antitumor activity similar to the parent compound but with a reduction in nonhematologic toxicity. The 1990s and beyond have brought important new agents into clinical practice, including the taxanes (paclitaxel and docetaxel), nontaxane microtubule-binding agents (epothilones), camptothecins (topotecan and irinotecan), nucleoside analogs (gemcitabine and capecitabine), alternative organoplatinums (oxaliplatin, satraplatin), an inhibitor of the proteasome complex (bortezomib), and an agent that binds to the minor groove of DNA, distinct from alkylating agents (trabectedin).
Attention has also been directed at alternative formulations of standard agents, including liposomal or polymer-based encapsulation, protein conjugation, nanoparticles, or lipid solubilization, to modify drug disposition, tumor targeting, and the potential for host toxicity. In addition, conventional agents have been utilized for regional (intraperitoneal) administration, prolonged intravenous infusion, continuous oral administration, high-dose therapy with hematopoietic progenitor cell support, and weekly low-dose therapy, each with the goal of improving the therapeutic ratio, but with a variable impact on host toxicity and clinical outcomes.
With the availability of multiple agents, each with a different molecular target, mechanism of action, pattern of resistance, and spectrum of host toxicity, we have also seen frequent utilization of multidrug combinations, particularly as primary therapy for advanced disease. The use of adjuvant therapy, including concurrent chemoradiation for early-stage disease, has been greatly expanded in selected clinical situations, with the result that a larger proportion of patients are exposed to chemotherapy at an earlier point in the natural history of their disease. Based on a combination of intrinsic and acquired factors, the majority of advanced tumors eventually demonstrate broad resistance to conventional cytotoxic chemotherapy, and there has been renewed interest in novel biologic and immunologic approaches with non-cross-resistant mechanisms. In addition, with improved understanding of the mechanisms associated with drug resistance, newer agents have been developed that may partially reverse resistant phenotypes through blockade of specific pathways, inhibition of DNA repair, or promotion of cellular apoptosis.
Molecular-Targeted Agents
Exploration of the biologic mechanisms associated with tumor invasion, metastasis, and angiogenesis has led to the development of inhibitors directed against metalloproteinases, adhesion molecules, and growth factors (including ligands and receptors). As basic research in tumor biology uncovers specific molecular targets, small molecules have been developed that modulate key pathways associated with tumorigenesis. These include blockade of gene products associated with specific activating (driver) mutations or genetic translocations, alterations in epigenetic factors (such as promoter methylation or histone modification), inhibition of protein tyrosine kinases associated with specific growth-factor receptors and downstream components of signal transduction, blockade of cyclin-dependent kinases and components of the mitotic apparatus associated with cell cycle progression, interference with posttranslational protein modifications (including proteasome function and catabolism), and modification of the of host immune response.
In general, these novel targeted agents exhibit toxicities that are distinct from conventional cytotoxic chemotherapy, often sparing proliferative compartments, such as bone marrow and mucosal epithelium. However, there is still the potential for serious toxicity, including drug interactions, alterations in hepatic or renal function, bleeding, thrombosis, pneumonitis, leukoencephalitis, and autoimmunity.
A Contemporary Challenge: Drug Shortages
Trends in drug development within the academic community and pharmaceutical industry have dramatically changed over the last 10 years, and we are now seeing an abundance of new molecular-targeted agents, with only a limited investment in conventional cytotoxics. Many of our most widely utilized standard chemotherapeutic agents have exceeded the period of patent protection and are now produced and distributed as generic compounds. With reductions in insurance reimbursement rates and the rising expense associated with manufacturing and quality control, the profitability of generic medications and the number of generic manufacturing facilities have declined. This has contributed to intermittent and chronic shortages of a number of compounds, including cisplatin, paclitaxel, 5-fluorouracil, leucovorin, bleomycin, polyethylene-glycosylated liposomal doxorubicin, antiemetics, and steroids. Consequences include greater costs to obtain drugs through alternate sources, omission of specific drugs from standard treatment regimens, and closure or delay of important clinical trials. Solutions to this international problem are complex, and it is likely that important shortages will manifest for a number of years to come. Information on current drug shortages can be obtained from the U.S. Food and Drug Administration (FDA) and the American Society of Health-System Pharmacists (ASHSP):
http://www.fda.gov/Drugs/DrugSafety/DrugShortages/default.htm
http://www.ashp.org/DrugShortages/Current
TUMOR BIOLOGY IN RELATION TO CHEMOTHERAPY
Tumor Growth and Cellular Kinetics
Many of the principles of modern chemotherapy are derived from knowledge of the growth characteristics of normal and tumor tissues. Each tissue has an innate capacity for growth that is regulated by internal and external factors. The growth characteristics of tumors differ from normal tissues, and the exploitation of these differences has provided the historical basis for utilization of radiation and chemotherapy. The cellular kinetics of normal tissues also explains many of the toxicities associated with chemotherapy. All normal tissues, particularly during fetal development and variably during adult life, possess the capacity for cellular division and growth.
The static population includes well-differentiated cells that arise from pluripotential fetal stem cells and rarely undergo cell division during adult life. Typical of this group are striated muscle, neurons, and nephrons, with oocytes representing a specialized subcategory. Damage to these cells can have long-term consequences and has prompted interest in stem cell biology.
The expanding population of normal tissues retains the capacity to grow, but in their adult state, they are normally quiescent. Under stress, especially after injury, a proliferative burst is followed by return to quiescence. Typical of this pattern of growth are the components of liver parenchymal tissue, including hepatocytes, bile duct epithelium, and vascular endothelium.
The renewing cell population is in a continuous proliferative state with ongoing cell division balanced by cell loss and terminal differentiation. Typical renewing populations include the bone marrow, epidermis, gastrointestinal epithelium, and spermatocytes.
The patterns of normal cell growth partially explain some of the toxic effects of cytotoxic therapy and why some tissues are commonly spared (8,9). Renewing cell populations with constant turnover are most sensitive to acute injury from conventional chemotherapy or irradiation. This is reflected by the frequent occurrence of dose-limiting bone marrow suppression, mucositis, and azoospermia during cytotoxic drug treatment, with relative sparing of nonproliferative compartments, such as brain, muscle, kidney, bone, and oocytes. However, even nondividing tissues can experience late chronic effects related to DNA damage.
Dysregulated growth of cancer cells occurs because of altered growth-factor signaling and/or disruption of checkpoint mechanisms that exist in normal cells. Despite a capacity for continuous growth, the actual process of cancer cell division is not more rapid than division in normal cells.
Programmed cell death, or apoptosis, has emerged as a major mechanism for regulating growth and development of tissues. Furthermore, certain oncogenes, like c–myc and bcl-2, and tumor-suppressor genes (antioncogenes), including Rb and TP53, are central to the regulation of apoptosis. Expression of these genes can alter the sensitivity of cancer cells to treatment with chemotherapy and radiation. For instance, overexpression of functional bcl-2 and nonfunctional p53 genes can render tumor cells resistant to a number of chemotherapeutic agents (10), suggesting that efforts to restore apoptotic signaling may improve chemosensitivity.
Emerging data have also described stem-like behavior in subpopulations of ovarian cancer cells, including dormant cells with a low mitotic index that exclude cytotoxic drugs from their cytoplasm and demonstrate increased resistance to chemotherapy (11,12). These stem-like cells are generally enriched during the repopulation that occurs following primary chemotherapy, and targeting of these distinct cell populations has become an important area of research.
Gompertzian Tumor Growth
During initial cell divisions, tumor growth seems to follow an exponential pattern. As the tumor grows larger, the rate of growth slows. This pattern of exponential growth with exponential growth retardation is known as gompertzian growth (Fig. 13.1). As the tumor mass increases, the time required to double the tumor volume also increases. The kinetic explanation for this apparent paradox is illustrated in Figure 13.2, which compares the number of cells in the tumor mass with the number of doublings (13). In accordance with gompertzian kinetics, exponential growth is not strictly maintained throughout the entire growth history. For example, if a skilled radiologist recognized a 0.5-cm tumor on a chest radiograph, or if a clinician palpated a 1-cm tumor mass, we might assume that the tumor had been detected quite early. In reality, the tumor has already undergone at least 30 doublings prior to detection, and this does not include any adjustment for ongoing cell loss.
FIGURE 13.1. Hypothetical gompertzian tumor growth curve. Exponential tumor growth with exponential growth retardation. The vertical axis is tumor volume, and the horizontal axis is time.
Limited information exists regarding the actual doubling times of human tumors in vivo (14,15), as summarized in Table 13.1. For this historical analysis, tumors were relatively circumscribed and serially measured by radiographic imaging, often as pulmonary metastases. It is clear that embryonal tumors, lymphomas, and mesenchymal tumors have shorter doubling times than adenocarcinomas or squamous cell carcinomas. In addition, metastases generally have faster doubling times than their corresponding primary lesions.
The model also suggests other conclusions of clinical relevance. First, metastatic spread may occur well before obvious evidence of the primary lesion. Second, at later stages of tumor growth, a small number of doublings can produce a marked change in tumor size, with an increased potential for adverse clinical consequences. For instance, a 1-cm mass (at least 30 prior doublings) becomes a 4-cm mass after just 2 more doublings.
Host-Tumor Interactions
Ultimately, dysregulated growth exceeds local resources, and the tumor becomes dependent on the manipulation of host angiogenic pathways for delivery of oxygen, access to nutrients, and removal of waste products. Tumors generally grow without induction of host lymphatics and are characterized by increased interstitial pressures as a consequence of disordered capillary proliferation with leaky vessels and accumulation of extracellular fluid. Together, these factors result in regional hypoxia, acidosis, and necrosis that can limit the effective delivery of chemotherapy and may protect viable tumor cells that are more distant from functional capillaries. One of the potential benefits observed with antiangiogenic therapy has been normalization of tumor vessels with reduced interstitial pressures and improved drug delivery (16).
FIGURE 13.2. Theoretical tumor doubling curve assumes exponential tumor growth. The vertical axis is the number of cells, and the horizontal axis is the number of doublings.
Cell Cycle Kinetics
The kinetic behavior of individual tumor cells is also important in understanding tumor growth. Figure 13.3 is a schematic view of the cell cycle. Cells can remain in a noncycling postmitotic compartment (G0) for extended periods of time, but retain the ability to reenter active cycling when triggered by growth factors or other local signals. The point of entry, or first gap phase (G1), can be of variable length and associated with diverse cellular activities, including protein and RNA synthesis, DNA repair, and cell growth. After passing the first checkpoint in G1, the cell enters the DNA synthetic phase (S), during which a complete copy of the cellular DNA is created through replication. The second gap phase (G2) provides another opportunity for checkpoint control before entering active mitosis (M), during which the nuclear membrane disappears and the chromosomes condense (prophase) and align (metaphase) in conjunction with the appearance of the mitotic apparatus, consisting of microtubules, centrioles, and the kinetochore. Mitotic alignment is associated with one final checkpoint prior to actual separation (anaphase), followed by dissolution of the mitotic apparatus (telophase) and creation of daughter cells through cytokinesis. The postmitotic period (G1) is variable, and cells can further differentiate, enter a noncycling state (G0), or initiate another cycle.
Doubling Times of Human Tumors |
Tumor Histology | Patients (n) | Doubling Time (Mean ± 2 SD, Days) |
Embryonal tumors (lung metastases) | 76 | 27 ± 5 |
Lymphomas | 51 | 29 ± 6 |
Malignant mesenchymal tumors | 87 | 41 ± 7 |
Squamous-cell carcinomas (lung metastases) | 51 | 58 ± 9 |
Squamous-cell carcinomas (primary tumors) | 97 | 82 ± 14 |
Adenocarcinomas (lung metastases) | 4 | 83 ± 12 |
Adenocarcinomas (primary tumors) | 34 | 166 ± 48 |
FIGURE 13.3. Phases of the cell cycle, beginning with M (mitosis) and proceeding through G1 (postmitotic phase), S (DNA synthetic phase), and G2 (premitotic phase). As G1 becomes progressively longer, it is known as G0.
Cell cycle events have important implications for the patient, tumor, and cancer therapist. Most chemotherapeutic agents disrupt DNA, RNA, or protein synthesis. Rapidly proliferating cells (i.e., short G1) are most sensitive to chemotherapy, whereas cells that slowly proliferate (i.e., G0 or long G1) are generally less sensitive. Nondividing cells, such as the differentiated elements of a mature teratoma, may occupy space and contribute to tumor bulk and symptoms, but are relatively insensitive to chemotherapy.
Cell cycle times may be estimated by performing labeled mitotic curves in vivo or in vitro, and appear to be relatively similar for many solid tumors, with cycle times ranging from 10 to 31 hours (17). Compared with the tremendous variation in human tumor doubling times, this is a relatively narrow range, suggesting that the primary reason for variations in clinical tumor growth cannot be ascribed to variations in the cell cycle.
Growth fraction and programmed cell death also influence the overall tumor growth rate and response to therapy. The growth fraction is the proportion of tumor cells that are actively cycling. Often, these proliferating cells are located proximal to small blood vessels. Growth fractions in human tumors are quite variable, ranging from 25% to 95%. Although it may seem paradoxical, cell loss is usually very high in human tumors, ranging from 70% to more than 95%, and small changes in cell loss could produce major changes in apparent tumor growth (18).
Log Cell Kill
In principle, the rational use of chemotherapy relies on basic concepts of cellular kinetics, but the translation from preclinical models to solid tumors has been challenging. In animals, the curability of transplanted tumors is inversely proportional to the tumor cell number and size, and timing of treatment initiation. In part, this is the result of important tumor-host interactions, such as the time required to establish a blood supply. However, this is also an illustration of first-order cell-kill kinetics, whereby a constant fraction of exposed cells is killed, rather than a constant number. Using first-order kinetics, a single treatment for a tumor weighing 1 g (approximately 109 cells) might yield 90% cell kill and would decrease the tumor population by only one log, leaving 108 viable cells. Without further treatment, the tumor would grow back at a constant rate, with only a modest delay in lethality. Only when the log cell kill is very large (>99%) and repetitive can chemotherapy be curative or capable of reducing residual disease to less than 104 cells. Certain cancers, such as choriocarcinoma, can be cured with a single application of a single drug. However, majority of cancers are intrinsically less sensitive and require multiagent chemotherapy over multiple cycles to achieve clinical benefit. Distribution of treatment over multiple cycles allows for host recovery while still achieving the cumulative cell kill required for tumor regression and cure.
Cell Cycle Specificity
Chemotherapeutic agents have complex mechanisms of action and can affect tumor cells through multiple pathways. Nevertheless, certain anticancer drugs are known to be proliferation-dependent and cell cycle-specific. Other drugs are cycle nonspecific, and are capable of killing in all phases of the cell cycle, generally without dependence on the proliferative rate. Examples of cycle-nonspecific drugs include alkylating agents, particularly nitrogen mustard, which are effective against a variety of solid tumors, including those with low growth fractions. Cell cycle–specific agents depend on the proliferative fraction of the tumor and phase of the cell cycle. A typical example is hydroxyurea, which inhibits ribonucleotide reductase. Not surprisingly, cell cycle–specific drugs tend to be more effective against tumors with a high proliferative rate and growth fractions. The interaction of specific cytotoxic agents with the cell cycle is summarized in Table 13.2.
PHARMACOLOGIC PRINCIPLES OF CHEMOTHERAPY
General pharmacologic principles should be considered by the physician in selecting individual drugs and managing their administration according to standards of dose and schedule. Many factors may influence the effectiveness and/or toxicity of chemotherapy, including absorption, distribution, metabolism, and excretion, as well as clinical complications, such as renal impairment, malnutrition, or drug-drug interactions.
Relationship of Chemotherapy Agents to Intracellular Targets and Cell-Cycle Progression |
Absorption, Distribution, and Transport
Drugs may be given orally, intravenously, intramuscularly, intraarterially, or intraperitoneally. Selection of the route of administration depends on solubility, requirements for drug activation, local tissue tolerance, feasibility for an individual patient, and optimal tumor drug exposure, which is estimated as the area under the concentration time curve (AUC) for the drug and active metabolites. The ultimate effectiveness of any chemotherapeutic agent depends on optimizing the AUC at critical tumor sites. However, there are no established techniques for noninvasive measurement of active drug concentration at these critical sites, and we are forced to extrapolate tumor drug exposure from preclinical models and plasma concentrations over time. Techniques such as magnetic resonance spectroscopy and positron emission tomography can potentially provide information on drug distribution and tumor metabolism, but they require a substantial investment in technology and specialized reagents.
The increasing utilization of oral chemotherapy and development of oral molecular-targeted agents has renewed interest in bioavailability in the setting of meals and other factors that can modulate local intestinal transport. In most cases, initial dose and schedule guidelines from FDA-approved package inserts reflect data from large phase 3 trials, rather than smaller studies that might examine dietary or drug interactions. For example, the bioavailability of one tyrosine kinase inhibitor could be increased over threefold in the setting of a high-fat meal (19), but the package insert recommends taking the drug on an empty stomach. With the high cost of chronic medication, it is apparent that there could also be substantial economic implications to these pharmacokinetic observations (20).
Some natural compounds, such as those found in grapefruit juice, can inhibit the cytochrome P450 isozyme CYP3A4 within the intestinal mucosa (21), as well as the drug efflux pump p-glycoprotein. Drugs that are substrates for either of these compounds can be more efficiently absorbed after ingestion of grapefruit juice, due to reduced local metabolism, leading to increased serum levels that may have clinical consequences, including cyclosporine, erythromycin, calcium channel blockers, and benzodiazepines.
The extent of drug binding to serum proteins, as well as physical properties, such as lipid solubility, diffusion, or molecular weight, may have an impact on tumor drug exposure. In addition, some areas of the body, particularly the brain, are actively protected from drug exposure and can serve as pharmacologic tumor sanctuaries, where small numbers of sequestered cancer cells may survive otherwise curative systemic therapy. Larger solid tumors eventually disrupt the blood-brain barrier, restoring access to conventional chemotherapy. In this manner, even drugs that are normally excluded from the central nervous system, such as carboplatin, can achieve dramatic reductions in metastatic disease (22).
In addition to achieving local delivery, most drugs must be internalized within tumor cells to achieve cytotoxicity. Internalization can be accomplished by passive diffusion, active transport, pinocytosis, or receptor-mediated endocytosis. Molecular targeting has also been utilized to enhance the delivery of conventional cytotoxic agents to tumors, based on expression of tumor-associated antigens, or the presence of specific receptors.
Many chemotherapeutic agents are lipophilic and highly protein bound in plasma, particularly to albumin. Commonly used agents with greater than 95% protein binding include cisplatin, paclitaxel, docetaxel, etoposide, and the active metabolite of irinotecan (SN-38). Agents with less protein binding include doxorubicin (75%), topotecan (35%), gemcitabine (10%), carboplatin (<5%), and ifosfamide (<5%). It is generally the unbound “free” drug that mediates toxicity, and any condition associated with variability in protein binding can have an impact on cumulative drug exposure. For example, the toxicity of chemotherapy is frequently accentuated in patients with poor nutritional status, which is associated with reduced protein levels and other metabolic changes (23). Docetaxel offers an interesting example for potential interactions, due to extensive binding to albumin, lipoproteins, and α-acidic glycoprotein (AAG), which has been correlated with changes in drug clearance, prompting further evaluation of optimized dosing (24).
Intraperitoneal Chemotherapy
Although most chemotherapy is administered by the systemic route, there are unique situations in which the regional use of chemotherapy has been studied, with intraperitonal administration a specific example. If primary tumors or their metastases are anatomically confined to specific organs or particular regions of the body, or if unique pharmacokinetic circumstances exist that favor regional clearance, there is a theoretical rationale for regional chemotherapy. For example, intraarterial drug administration has been studied in cervical cancer, localized recurrence of rectal carcinoma, and head and neck cancer. Local complications are potentially serious, including arterial thrombosis, wound slough, lymphedema, and osteonecrosis caused by the shared arterial blood supply between the tumor and neighboring normal tissues. While high response rates can be achieved in selected patients, larger randomized trials are needed to evaluate overall risks and long-term outcomes.
Intracavitary chemotherapy has been used for tumors confined to the peritoneum, pleura, or pericardium. The rationale for this approach is based on the fact that clearance from a body cavity is delayed compared to the systemic circulation, achieving more prolonged exposure to higher regional concentrations of active agents. This technique has been most extensively studied in ovarian cancer, with evaluation of many agents, including 5-fluorouracil, doxorubicin, cisplatin, carboplatin, cytarabine, melphalan, etoposide, and paclitaxel (25,26
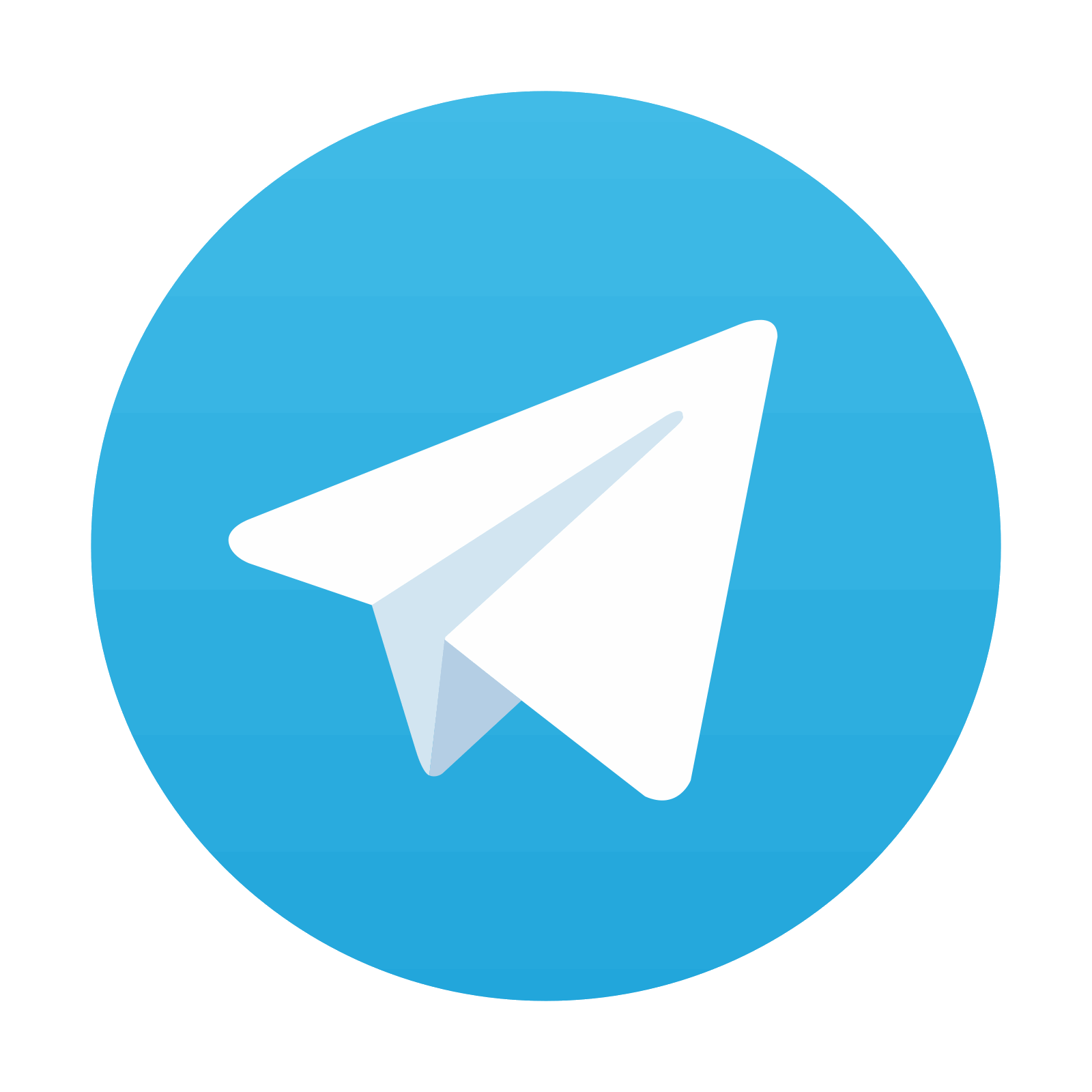
Stay updated, free articles. Join our Telegram channel
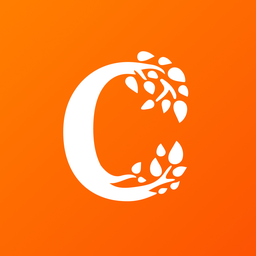
Full access? Get Clinical Tree
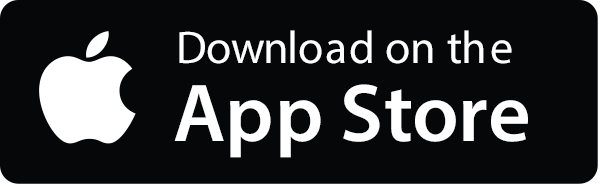
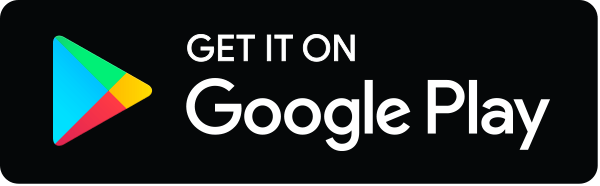