Name (year)
Formula
Ref.
Warsof (1977)
log10BW = −1.599 + (0.144 × BPD) + (0.032 × AC) − (0.000111 × BPD2 × AC)
[3]
Shepard (1982)
log10BW = −1.7492 + (0.166 × BPD) + (0.046 × AC) − [(2.646 × AC × BPD)/1000]
[4]
Hadlock (1985)
log10BW = 1.335 − (0.0034 × AC × FL) + (0.0316 × BPD) + (0.0457 × AC) + (0.1623 × FL)
[5]
Aoki (1985)
EFBW = (1.25647 × BPD3) + (3.50665 × FTA × FL) + 6.30994
[6]
Shinozuka (1987)
EFBW = (1.07 × BPD3) + (3.42 × APTD × TTD × FL)
[7]
Modified Shinozuka (2000)
EFBW = (1.07 × BPD3) + (0.30 × AC2 × FL)
[8]
When an abnormality is detected during prenatal US examination or when there is an increased risk for neurodevelopmental disabilities, MRI is the next imaging choice for further examination. Fetal MRI can reveal abnormalities of the central nervous system that are not apparent on prenatal US in approximately 20% of cases [17]. If an atrial width of ≥10 mm is detected at the glomus of the choroid plexus on an axial ultrasound image at the level of the thalamus, it is considered to represent ventriculomegaly [18]; thus, an MRI scan would be performed for further diagnosis. Fetal MRI reveals additional abnormalities in up to 50% of ventriculomegaly cases identified in US images [19, 20]. Commonly associated anomalies include agenesis of the corpus callosum, migrational anomalies, and ventricular hemorrhage. Holoprosencephaly (HPE) can also be detected by ultrasound and MRI; however, milder cases of lobar HPE can be difficult to detect considering the wide spectrum of HPE symptoms. Cortical malformations are characteristics of target diseases in fetal MRI, although the normal smoothness of the cortex in the second trimester makes it difficult to be distinguished from that in migrational disorders [21]. Intracranial tumors are quite rare and can be detected by fetal MRI, mostly as a supratentorial lesion with mixed/high signal intensity on T2-weighted sequences.
MRI is also effective for other congenital anomalies. For congenital diaphragmatic hernia (CDH), which occurs in 1 in 4000 live births, fetal MRI is superior to US in distinguishing the subtypes of CDH [22]. The analysis of fetal lung volume is important in CDH for evaluation of prognosis, and MRI is commonly used to calculate the volume during evaluation. This function of MRI can also be applied to other diseases involving lung mass, such as pulmonary sequestrations, congenital pulmonary airway malformations, and, very rarely, neoplasms. Furthermore, MRI is used in the diagnosis of ventral abdominal wall defects such as gastroschisis and omphalocele. Axial T2-weighted sequences are the most helpful for revealing defects in the abdominal wall and the position of the umbilical cord, whereas T1-weighted sequences are useful in tracing the bowel [23, 24]. Musculoskeletal system evaluation is still one of the challenging aspects of using fetal MRI. Diffusion tensor imaging (DTI) has recently been applied in clinical MRI, and muscle fibers can be imaged by DTI in adults [25]. Although fetal muscle imaging using DTI has started only in experimental animals [26], it will be applied for fetal MRI in the near future.
Despite the risk to the fetus by exposure to ionizing radiation, X-ray computed tomography (CT) plays an important diagnostic role during the prenatal period, especially for fetal skeletal diseases (SDs). The sensitivity to SDs in ultrasonography screening is limited, ranging between 40 and 60% [27, 28], and diagnostic three-dimensional ultrasonography offers a better sensitivity of around 80% [29–31]. MRI is no more effective than ultrasonography for further diagnosis of SDs [32]. In recent years, fetal skeletal CT has been used for visualizing the fetal skeleton [33, 34]. A low-dose CT protocol with 3D reconstruction has been suggested for decreasing the adverse effects of X-ray, and fine images can be obtained for accurate diagnosis [32, 35].
12.1.2 Autopsy Imaging of Human Embryos and Fetuses
For dead human embryos and fetuses, additional imaging modalities can be applied. Classically, solid reconstruction and fine drawing have been the primary approaches used, for example, the wax plate technique using serial histological sections of human embryos was the first 3D morphological imaging technique developed by Gustav Born [8]. Recently, the 3D reconstruction of serial sections is performed using computer graphic methods; therefore, the 3D reconstruction becomes easier and quicker than before [36]. The 2D image stacks generated from serial sections have a high resolution; however, they have issues of section registration and distortion. A solution to this problem is by using episcopic fluorescence image capture (EFIC), a novel imaging modality for the generation of high-resolution 3D reconstructed images [37]. In EFIC imaging, tissue autofluorescence is used to image the block face prior to cutting each section. Although the samples are sliced and lost during the procedure, the optical resolution of EFIC has been reported to reach approximately 5–6 μm [38].
MRI is a useful imaging modality not only for living prenatal embryos and fetuses but also for dead embryos and fetuses as autopsy imaging. Since it takes a longer time to capture images, images with a higher resolution can be obtained. The imaging time for high-resolution images ranges from several hours to several days. MR devices should be selected depending on the sample size; MR microscopy, clinical MRI, and experimental MRI are suitable for small-sized embryos, larger fetuses, and embryos or fetuses with an intermediate size, respectively (Fig. 12.1a–c) [39, 40].

Fig. 12.1
(a) MR microscopy at 2.34 T; digital resolution is 40 μm3. (b) Clinical MRI at 3 T; digital resolution is 200 μm3. (c) Experimental MRI at 7 T; digital resolution is 35 μm3. (d) Conventional (absorption-contrast) X-ray CT; digital resolution is 200 μm3. (e) Phase-contrast X-ray CT; digital resolution is 6.5 μm3
X-ray imaging is also used for dead embryos and fetuses. Since there is no need to consider the influence of radiation exposure, it is possible to devote a longer time to imaging. Conventional (absorption-contrast) X-ray CT (cCT) is used for fetal skeletal imaging (Fig. 12.1d). Phase-contrast X-ray CT (pCT) is another method of X-ray imaging [40]. Owing to the characteristics of X-rays as electromagnetic waves, phase-contrast X-ray imaging is able to visualize the phase shift of X-rays passing through the samples and reconstruct 2D or 3D images of the samples in combination with CT. An embryo or early fetus is mostly composed of soft tissue because of the absence of a bony structure and thus is suitable for pCT (Fig. 12.1e).
Ultrasonography of living embryos and fetuses is now commonly performed, and many malformations can be diagnosed during the early prenatal period. In cases of pregnancy termination, not all of the aborted fetuses are dissected and pathologically diagnosed because it is technically difficult to dissect small fetuses. The imaging modalities presented here can be used for autopsy imaging of embryos and fetuses in the future. If clues for diseases the fetus may have had can be identified by imaging, the appropriate genetic tests can be performed, and a final accurate diagnosis can be obtained. With the final diagnosis, parents would have sufficient information about their lost pregnancy and can receive appropriate genetic counseling for the next pregnancy.
The imaging modalities described in this section are summarized in Fig. 12.2. The appropriate modalities for imaging of dead embryo or fetus should be used depending on the period of the pregnancy.

Fig. 12.2
Relationship between sample size and 3D imaging techniques for the human embryo, fetus, and newborn. pCT phase-contrast X-ray computed tomography, cCT conventional (absorption-contrast) X-ray computed tomography, EFIC episcopic fluorescence image capture, MRM magnetic resonance microscopy, clinical MRI magnetic resonance imaging for routine clinical use, clinical USG ultrasonography for routine clinical use
12.2 Genetic Analysis of the Human Embryo and Fetus
The amniotic fluid, chorionic villi, and umbilical cord blood have been used for genetic analyses of human embryos and fetuses. Recently, DNA fragments derived from villus cells have been identified in the maternal blood [41, 42], and the genetic information of the fetus could be determined from maternal blood analysis; this approach is called noninvasive prenatal genetic testing (NIPT). In comparison with maternal serum analysis, NIPT for aneuploidy has considerably higher sensitivity and specificity [43]. However, NIPT is a screening test with the potential for false-positive and false-negative results because cell-free DNA (cfDNA) could also be derived from multiple sources such as placental mosaicism, maternal conditions including cancer, or fetal and/or maternal copy number variation (CNV) [44].
The cell samples obtained from the amniotic fluid and chorionic villi are used for both screening and diagnostic tests. Several laboratory techniques can be used for prenatal genetic diagnosis. Traditional karyotype analysis is most commonly used to examine cells obtained by chorionic villus sampling (CVS) and amniocentesis (AC). This method is appropriate for the diagnosis of aneuploidies and large rearrangements. The diagnostic accuracy of traditional karyotype analysis is greater than 99% for aneuploidy and chromosomal abnormalities larger than 5–10 Mb [45]. Fluorescence in situ hybridization (FISH) analysis can detect specific chromosomes or chromosomal regions by using fluorescently labeled probes. The turnaround for FISH results (usually within 2 days) is faster than that for conventional karyotyping results (7–14 days, including the cell culture period). FISH is commonly used as a screening panel for chromosomes 13, 18, 21, X, and Y. It is considered a screening test because false-positive and false-negative results have been reported with FISH [46–48]. Therefore, clinical diagnosis using FISH results should be supported with other clinical and laboratory analyses, such as abnormal ultrasonography, positive screening test using maternal serum and/or soft markers, or confirmatory traditional metaphase chromosome analysis or chromosomal microarray analysis (CMA), as described in the next paragraph.
CMA can detect small chromosomal aneuploidies that cannot be identified by conventional karyotyping [49]. The duplicated or deleted regions of DNA are called CNV. CMA can be performed without cell or tissue culture; thus, the results are obtained in approximately 3–7 days. Since CMA can also be performed with nonviable cells, which are not suitable for conventional karyotyping analysis, cases of fetal death or stillbirth can be examined by this technique [49]. CMA can identify nearly all abnormalities except for balanced translocations and triploidy. When CMA is compared with conventional karyotyping in the detection of structural abnormalities by prenatal ultrasonography, approximately 6% of the fetuses were identified with chromosomal abnormalities by CMA; however, conventional karyotype analysis presented normal results [50, 51]. Therefore, CMA should be the primary test if a structural abnormality is detected by fetal ultrasonography, as recommended by the American Congress of Obstetricians and Gynecologists (ACOG) [49].
In the late 1980s, single-gene disorders were diagnosed using fetal samples. At first, prenatal diagnosis of β-thalassemia was performed using amplified fetal DNA [52], and then the number of diagnosable diseases or genes has increased. Whole-genome sequencing using DNA samples from the amniotic fluid was performed in the next-generation sequencing (NGS) era [53]. Whole-exome sequencing (WES) is also a choice for fetal genetic analysis because coding exons sequenced in WES are only 2% of the genome but contain 85% of disease-coding mutations. Prenatal WES using fetal blood samples has been performed since 2013 [54]. In the late 2000s, massive parallel sequencing (MPS) using NGS opened the way to NIPT [55]. Now, NIPT for aneuploidy is widely used in the world [56], and some fetal single-gene diseases can also be detected using cell-free fetal DNA (cffDNA) obtained from maternal blood [57, 58]. Although the number of diseases that can be detected using cffDNA is gradually expanding, cffDNA analyses are screening tests and do not replace diagnostic testing, as mentioned in the guidelines of professional societies [59–64].
12.3 Ethical Issue of Prenatal Diagnosis
Prenatal diagnosis involves certain ethical issues, and recent progress in fetal genetic testing also presents additional ethical challenges. The four principle-based ethics are (1) respect for autonomy, (2) beneficence, (3) non-maleficence, and (4) justice. All women have reproductive rights to make the final reproductive decision following appropriate counseling or advice based on these principles. Respect for autonomy requires that parents are given accurate information so they can make a properly informed decision [65]. For example, the specific steps in genetic counseling for pre-NIPT include pretest education, counseling, and informed consent; the screening or testing procedure; a laboratory component that includes test interpretation; and, finally, the disclosure of results to the patient within a context that includes the appropriate education, counseling, and follow-up [64]. Moreover, since termination for fetal abnormality can have long-lasting psychological consequences, identifying women vulnerable to poor psychological adjustment and promoting coping strategies associated with lower levels of grief may be beneficial [66]. In Japan, prenatal genetic screening and testing are offered to women whose child is at risk for severe child-onset diseases and/or chromosomal abnormalities, in accordance with criteria provided by Japanese professional societies. Some people claim that the detection of congenital anomalies should reduce cases of disabilities; the response of the WHO to this claim is that the availability of genetic tests must not be allowed to create an illusion that most disabilities are preventable and therefore unacceptable to society [67].
In the near future, we should be able to detect the whole genomic information, epigenetic condition, and molecular structure of a fetus with ease. The analysis results would contain information about not only life-threatening diseases but also the general phenotype. Furthermore, fetal therapy and genome editing will be practically performed. In the context of prenatal diagnosis, we should consider our ethical responsibilities to two patients: the mother and the fetus. The more we possess, the harder quandaries lead.
References
1.
Rao R, Platt LD. Ultrasound screening: status of markers and efficacy of screening for structural abnormalities. Semin Perinatol. 2016;40(1):67–78.CrossRefPubMed
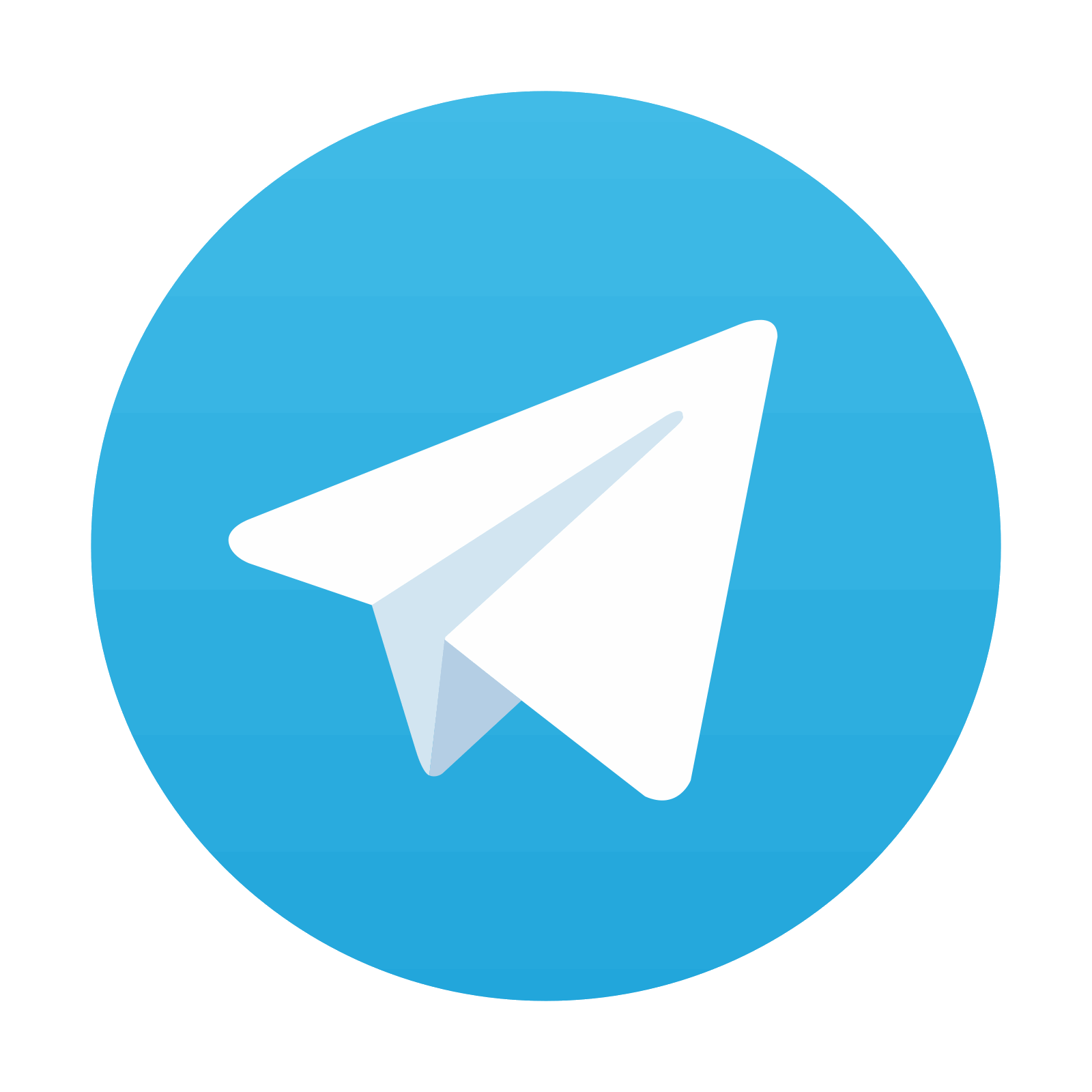
Stay updated, free articles. Join our Telegram channel
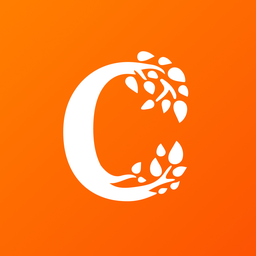
Full access? Get Clinical Tree
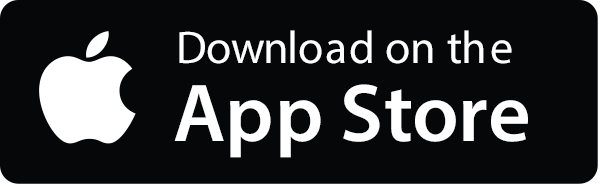
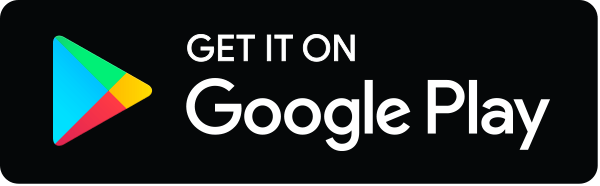