Fig. 3.1
Association of pericytes and endothelial cells. a Schematic representation of endothelial cells (blue) and mural cells (pink). Vascular smooth muscle cells cover larger vessels such as arterioles. In capillaries, pericytes are found on the abluminal surface of endothelial cells embedded in the vascular basement membrane. b Immunofluoresent micrograph of mouse retinal vasculature with endothelial cells stained with BSL-I (green) and pericytes stained with an anti-NG2 antibody. c Electron micrograph showing a cross-section of a central nervous system capillary. The endothelial cell (e) forms a tube for the passage of blood, and the pericyte (p) is situated on the abluminal surface of the endothelial cell. d Electron micrograph demonstrating the interaction of pericytes (p) with endothelial cells (e) in a mouse cerebral capillary. Pericytes are embedded in the vascular basement membrane with intermittent junctional contacts with the endothelial cell
Although much has been learned about the cell biology, development, and function of pericytes in the last 140 years, considerable mystery persists regarding the role of these cells in regulating tissue development, vascular function, homeostasis, and response to injury and disease. Much of the difficulty in studying pericytes comes from the fact that pericytes lack clearly defined histological and molecular criteria. While many researchers identify pericytes based on their proximity to microvascular endothelial cells , many other cell types in different tissues are associated with the abluminal surface of endothelial cells, including vSMCs, macrophages, fibroblasts, and epithelial cells. The lack of distinct molecular markers for pericytes has hindered the precise identification of each cell type, and thus there is often ambiguity regarding the cell population being studied. The most stringent definition of a pericyte requires that a cell is embedded within the vascular basement membrane [5]. However, studies suggest that pericytes exhibit dramatic plasticity, including cell proliferation, morphogenesis, and migration [1]. These processes that occur in response to injury and disease include breakdown of the extracellular matrix (ECM) and movement away from the vascular basement membrane. Therefore, defining pericytes strictly based on their localization in the basement membrane is too limited and fails to reflect these dynamic properties. Furthermore, there is evidence suggesting the heterogeneity of pericyte populations in different organs, and even within the same organ, further obscuring the identification of this cell type .
In the upcoming years, the identification of novel pericyte-specific molecular markers, and the generation of genetic tools to label pericytes in vivo, as well as new imaging techniques to recognize pericyte cell morphology and localization, may provide a better definition for this mysterious cell type.
3.2 Cell Biology of Pericytes
3.2.1 Cell Morphology
Pericytes are associated with the abluminal surface of microvessels, including capillaries and postcapillary venules throughout the body. These cells adhere to the abluminal surface of endothelial cell tubes, and are embedded in the vascular basement membrane [1, 6, 7]. Pericytes commonly extend long, branching cytoplasmic processes along the abluminal surface of the blood vessels that can stretch across multiple endothelial cell bodies (Fig. 3.1). The cell body of pericytes is often found at branch points of the microvascular network.
On capillaries, pericytes have a rounded cell body that contains a few primary cellular processes branching into perpendicular secondary processes that connect with endothelial cells . In postcapillary venules, pericytes display a more stellate pattern, with a flattened cell body and many thin, branching cellular processes [7].
Electron microscopy (EM) has identified that pericytes possess a discoid nucleus, few cytoplasmic organelles, and abundant plasmalemmal vesicles. Serial section EM has demonstrated that these vesicles interconnect to form a continuous network associated with the parenchymal-facing membrane of the pericyte [8]. The pericyte cell body is rich in cytoskeletal elements, such as intermediate filaments composed of desmin and vimentin extending into the primary extensions, and microtubules extending into both the primary and secondary cellular processes [1]. In addition, bands of actin, myosin, and tropomyosin are situated beneath the plasma membrane, indicating these cells are capable of contraction [9]. Although most of the cell body of the pericyte is separated from the endothelial cell by the vascular basement membrane, pericyte processes are observed to contact the endothelial cells in so-called peg-and-socket junctions [10–12].
As noted, one of the major challenges in studying pericytes comes from the lack of defining molecular markers. There is no molecular marker that is unique to pericytes. Recent findings demonstrating molecular heterogeneity of pericytes in different tissues and the ability of pericytes to change their morphology and molecular composition in response to injury, disease, and cell culture, further complicate the identification of pericytes. One commonly used molecular marker is platelet-derived growth factor receptor (PDGFR)-β, a receptor tyrosine kinase that is required for the recruitment of pericytes to the vascular tubes. However, PDGFRβ is also expressed in other cell types, including neural cells, cardiomyocytes, and fibroblasts [13, 14]. In addition, markers such as NG2, Anpep (CD13), desmin, RGS5, Abcc9, Kcnj8, Dlk, and Zic1 have all been utilized to identify pericytes, yet all lack specificity [1, 15–19].
3.2.2 Heterogeneity of Pericytes
Recent evidence suggests that there is remarkable heterogeneity of pericytes at different levels of the vascular tree, especially notable when comparing pericytes on postcapillary venules with pericytes on capillaries. Postcapillary venules have a greater number of flattened pericytes compared with capillaries, with thinner cellular processes and a greater extent of overlapping branches [20, 21].
In addition, there are clear differences between pericytes in various organs, as well as distinct subpopulations within an organ. These differences may indicate the importance for the vasculature to meet the unique requirements of each tissue and organ in the body .
Pericyte cellular density and extent of coverage of the vascular tube differ in various tissues. For example, central nervous system (CNS) capillaries have the largest number of pericytes, with an endothelial to pericyte ratio between 1:1 and 3:1, whereas skeletal muscle shows a pericyte:endothelial cell ratio of 100:1 [22]. However, it should be noted that such metrics are imprecise since there are no reliable markers for counting the exact number of pericytes in each tissue. The extent of coverage by pericytes of the external surface area of the vascular tube also varies and has been estimated to be 11 % in cardiac muscle, 21 % in skeletal muscle, 22–32 % in the cerebrum, and 41 % in the retina [23]. The density and vascular coverage of pericytes inversely correlates with the permeability of the vessel as well as the rate of endothelial cell turnover. These observations have led to the hypothesis that pericytes may be critical for endothelial stability and limiting permeability. Indeed, experiments using genetically engineered mice that lack pericytes have confirmed a role for pericytes in these processes [17, 24]. Pericytes in different tissues show variable levels of granularity, an indication of the abundance of cytoplasmic lysosomes. All cerebral pericytes are granular, a property that is increased following disruption of the blood–brain barrier (BBB) [5] .
Pericyte populations within specific tissues have evolved unique morphological and physiological properties, many of which were identified initially by different names. For instance, stellate cells represent hepatic pericytes [25]. These cells are localized between endothelial cells of the sinusoidal capillaries and the parenchyma in the space of Disse [26] and are not embedded within the vascular basement membrane as sinusoidal capillaries have incomplete basement membranes and thus do not comply with classical definition of pericytes. Stellate cells contain cellular protrusions that adhere both to the endothelium and hepatocytes, and express desmin, glial fibrillary acidic protein, neural cell adhesion molecule, synaptophysin, nestin, and smooth muscle actin (SMA) when activated. These cells possess functions unique to the liver as they are involved in vitamin A storage, liver development, regeneration, and fibrosis (discussed in the ‘Regulation of Tissue-Specific Properties’ section) [27] .
Evidence suggests that even within a given organ, there are different pericyte populations based on their localization. For instance, in the kidney there are two types of pericytes: the tubulointerstitial capillaries are covered by what are thought to be classical pericytes, whereas the glomerulus has specialized pericytes termed mesangial cells [28, 29]. Mesangial cells make up 30 % of all glomerular cells and play an important role in capillary tuft morphogenesis and the regulation of glomerular filtration (discussed in the ‘Regulation of Tissue-Specific Properties’ section). These cells express many classical pericyte markers, such as PDGFRβ and CD90, and are thus thought to be locally specialized pericytes [29]. In the cochlea, pericytes on vessels in different regions have different morphologies and express different molecular markers, depending on their localization on the capillary bed. Pericytes on vessels in the spiral ligament express α-SMA, desmin, and tropomyosin, whereas pericytes on vessels of the stria vascularis express only desmin [30].
In addition, different subpopulations of pericytes appear to be interspersed along the vascular tree. In the CNS, EM has been used to identify four subclasses of pericytes based on morphology of cellular processes and location of the nucleus. These different morphologic categories include (i) pericytes with broad cellular processes that are continuous with the surface of the endothelial cells; (ii) pericytes with thin, finger-like cellular projections confined to specific regions of the endothelial tube; (iii) pericytes that extend processes longitudinally along the axis of the capillary; and (iv) pericytes in which the cell body and processes are retracted from the endothelial tube [6]. These features may define specific subsets of pericytes, or may indicate unique pericyte characteristics at specific moments, including migration along the vessel during angiogenesis or migration away from the vessel in response to injury and disease. Furthermore, several studies have identified subtypes of pericytes in the lung, skin, kidney, and CNS that differ in their capacity to proliferate and form a scar tissue after tissue injury (see ‘Regulation of Injury/Disease’ section) .
Furthermore, the location of pericytes along the vascular tube varies. For instance, in the choriocapillaris of the eye, pericytes are positioned distal to localized oxygen transport [31] and, in bovine, lung pericytes reside near endothelial cell junctions [32]. In muscle, pericytes have been observed to cover histamine-induced gaps in the vasculature [33]. These data suggest that the location of pericytes within the vasculature may be important for specific vascular function within a tissue or organ.
3.2.3 Cell Adhesion to Endothelial Cells
Although pericytes line the outer surface of the endothelial cell tube, most of the pericyte cell body is not in contact with endothelial cells but is separated by the vascular basement membrane. However, there are discrete points where pericyte cellular processes contact endothelial cells to form cellular adhesions; it is estimated that a single pericyte can form up to 1000 adhesion contacts with endothelial cells [1].
The main type of cellular junction formed between pericytes and endothelial cells are described as ‘peg-and-socket’ junctions. In these junctions, cellular protrusions from the pericyte (pegs) adhere to invaginations (sockets) in the endothelial cells. These junctions are thought to be mediated by N-cadherin interaction based on the observation that N-cadherin expression has been visualized at points of pericyte–endothelial cell interactions, and the addition of N-cadherin blocking antibodies leads to defective pericyte adhesion to the vascular tube [34]. Further evidence suggests that transforming growth factor-β (TGFβ) signaling is required for adhesion since disruption of Smad4 leads to loss of pericyte–endothelial cell interactions due to diminished N-cadherin expression [35] (discussed in the ‘Vascular Recruitment’ section).
In addition, several other types of cellular adhesions have been visualized between pericytes and endothelial cells. Adhesion plaques have been identified and are characterized as membrane contacts in which microfilament bundles are adjacent to the pericyte membrane, and an electron dense endothelial cytoplasm is adjacent to the endothelial membrane [36]. In brain microvessels, fibronectin has been observed at the adhesion plaques, suggesting that this could be a critical mechanical linkage, perhaps involved in vessel contractility. Several studies have suggested that gap junctions allow for electrical coupling of endothelial cells and pericytes. Functional gap junctions have been observed between endothelial cells and pericytes in vitro using a co-culture paradigm [37], and gap junctions have been visualized between endothelial cells and pericytes in vivo [38]; however, it has yet to be determined whether there is electrical coupling of endothelial cells and pericytes by these gap junctions in vivo. Although not fully characterized, tight junctions have been observed between endothelial cells and pericytes in vivo [12, 32].
3.2.4 Interaction with the Extracellular Matrix
Pericytes are embedded in the vascular basement membrane and thus share an ECM with CNS endothelial cells. Several studies using cell purification and microarray analysis have identified that pericytes express many different components of the EMC, including collagen subunits, laminin subunits, vitronectin, and asporin. Time course studies of vascular development suggest that pericyte recruitment to the endothelial tube correlates with the onset of vascular basement membrane deposition. Endothelial cell–pericyte co-cultures suggest that pericytes can also induce the expression of ECM components, such as nidogen and collagen, by endothelial cells [39]. Furthermore, disruption of platelet-derived growth factor-B (PDGF-B)/PDGFRβ signaling, which inhibits the recruitment of pericytes to endothelial cells, causes marked disruption in the vascular basement membrane and leads to protrusions of endothelial cells into the CNS parenchyma [15]. Taken together, these data suggest that recruitment of pericytes to endothelial cells is critical for the deposition of EMC, which is produced by both cell types .
Additionally, it appears that the vascular basement membrane is critical to the recruitment and adherence of pericytes to the endothelial cells. RNA interference (RNAi)-mediated inhibition of tissue inhibitor of metalloproteinase 3 (TIMP-3) leads to increased matrix metalloproteinase disruption of the ECM and causes an increased vessel diameter and loss of endothelial cell–pericyte interactions [39]. One of the critical functions of the ECM is to localize the PDGF-B signal that is required for the recruitment of pericytes to the endothelial cells. Mutation of the ECM retention motif of PDGF-B leads to a poor pericyte recruitment to the endothelial tube [40] (discussed in the ‘Vascular Recruitment’ section).
While the ECM is critical to mediate endothelial-pericyte interactions during angiogenesis and adult tissue homeostasis, alterations in the basement membrane can lead to loss of pericyte–endothelial cell interactions during injury and disease. For instance, following a stroke there is a degradation of the vascular basement membrane mediated by matrix metalloproteinases (MMP)-2 and MMP-9, which coincides with the migration of the pericytes away from the endothelium [41, 42]. This can also be observed during tumor angiogenesis where the formation of new blood vessels involves the degradation of the vascular basement membrane and often results in endothelial cells poorly covered in pericytes .
A recent study suggests that pericyte–ECM interaction is an important regulator of the differentiation state of pericytes. In the CNS, loss of astrocyte-derived laminin was shown to drive expression of contractile proteins in pericytes, and pericyte interaction with laminin-111 via α2 integrins was required to maintain the noncontractile phenotype [43].
3.3 Cell Development
3.3.1 Cell Lineage
The few studies that have investigated the developmental origin of the mural cells covering microvasculature (i.e. pericytes) suggest a common origin for pericytes and vSMCs (reviewed by Armulik et al. [1]). However, mural cells have different developmental origins depending on which vascular bed they are located. The first blood vessels to develop in the embryo proper are the paired dorsal aortae that arise from the trunk. Initially, mural cells in the floor of the dorsal aorta are derived from splanchnic mesoderm, and mural cells on the roof are derived from sclerotome, similar to endothelial cells (reviewed by Sato [44]). Later during development, splanchnic-derived mural cells are replaced by sclerotome-derived cells [45]. Mural cells of the ascending and arch portions of the aorta, ductus arteriosus, the brachiocephalic, right subclavian arteries, and common carotid arteries arise from neural crest (reviewed by Majesky [46]). Similarly, mural cells in the heart exhibit several distinct developmental origins. Specifically, vSMCs of the proximal and major anterior coronary arteries arise from neural crest, whereas the rest derive from the proepicardial organ and epicardium. Coronary vein vSMCs have an atrial cardiomyocyte origin (reviewed by Riley and Smart [47]). Neural crest gives rise to vascular mural cells in the head region, CNS, and thymus (reviewed by Armulik et al. [1]). Mural cells in the gut, liver, and lung are derived from mesothelium (reviewed by Armulik et al. [1]) in contrast to those in the limbs and peritoneum, which are derived from the sclerotome [48]. It is unknown whether the different developmental origins of the mural cells in different vascular beds give rise to tissue-specific functions of these cells.
3.3.2 Differentiation
The transcriptional regulation of vSMC differentiation is relatively well understood, with vSMC-selective gene expression achieved by a unique combination of multiple ubiquitously expressed or selective factors (reviewed by Alexander and Owens [49]). Several signaling pathways (Notch, TGFβ [discussed 3.3.3], Wnt) have been shown to be important for initial vSMC differentiation surrounding the aorta [53–52] .
The transcriptional mechanisms that control pericyte differentiation remain elusive. However, a recent report by Siegenthaler et al. demonstrates that genetic ablation of Foxc1 in pericytes leads to pericyte and endothelial cell hyperplasia, thus underscoring its role as an important regulator of pericyte maturation [52]. During development, several pericyte markers (e.g. RGS5, endosialin, NG2, desmin) show dynamic expression. The expression of RGS5, endosialin, and NG2 in pericytes is selectively decreased as the vasculature matures, whereas desmin expression increases [54–57]. According to a recent study, Dll4 and PDGF-B signaling induces the differentiation of pericytes from myoblasts [58]. It is not known to what extent PDGF-B/PDGFRβ and Notch signaling regulate pericyte differentiation during angiogenesis; however, Notch signaling has been shown to regulate PDGFRβ expression on vSMCs [59]. Additionally, Notch signaling in mesangial cells (specialized pericytes in kidney glomeruli) was shown to be necessary for specification of mesangial cell precursors that express a high level of PDGFRβ [60]. Of note, increased PDGFRβ signaling in brain pericytes results in higher pericyte proliferation and a less mature phenotype [61]. This suggests that the PDGFRβ activity that is required for pericyte recruitment may be needed to be switched off for pericyte maturation. It is interesting that brain pericytes have been suggested to differentiate within the mesenchyme that surrounds the telencephalon before entering the CNS [62].
3.3.3 Vascular Recruitment
Several well-characterized signaling pathways are critical for pericyte recruitment during angiogenesis, including the PDGF-B/PDGFRβ and TGFβ signaling axes (Fig. 3.2). In addition, other pathways that regulate pericyte recruitment are recognized, although largely in the context of pathological conditions (Fig. 3.2). It should be underlined that, based on current knowledge, no universal signaling pathway seems to regulate pericyte recruitment to all organ vascular beds during development, injury, or pathological angiogenesis . Most likely, pericyte recruitment to the developing vasculature in a given organ is guided by a combination of signaling pathways that is specific to each organ.

Fig. 3.2
Signaling pathways important for pericyte recruitment, differentiation, and vessel stabilization. Multiple ligand receptor complexes mediate pericyte recruitment to the endothelium during angiogenesis. Some of these pathways (SDF1α/CXC4R, HB-EGF/ErbB) appear relevant only in pathological angiogenesis. A ligand–receptor pair is indicated by the same color. Modified from Armulik et al. [1]
Signaling Axis: PDGF-B/PDGFRβ
Many studies have confirmed the importance of the PDGF-B/PDGFRβ signaling axis for pericyte recruitment during development and pathological angiogenesis. The importance of PDGFB in vascular development and pericyte recruitment was somewhat unexpected. In fact, PDGFB, the first oncogene to be cloned and sequenced, was recognized as a growth factor for mesenchymal cells. However, the critical role of PDGF-B in pericyte recruitment to the developing microvasculature was demonstrated by the genetic ablation of Pdgfb. Mice lacking Pdgfb, or its receptor Pdgfrb, showed almost identical phenotypes, with late embryonic lethality caused by dysfunctional vasculature [17, 63]. During angiogenesis, outgrowing endothelial cells express high levels of PDGF-B. Subsequently, secreted PDGF-BB is mobilized to the ECM adjacent to the endothelium. Pericytes, which express PDGFRβ, respond to the PDGF-BB gradient by proliferation and migration along the developing vasculature (reviewed by Armulik et al. [1]). The dependency of pericyte recruitment on PDGF-B/PDGFRβ signaling is organ-specific. Pdgfb or Pdgfrb knockout embryos lack pericytes in organs such as the brain, kidney, skin, and muscle, but not in the liver and thymus.
The binding of PDGF-BB to the ECM is critical for the bioavailability of this signaling molecule. The importance of localized presentation of PDGF-BB in pericyte recruitment was demonstrated in genetically-modified mice that express PDGF-B protein lacking the so called ‘retention motif’, thus preventing mobilization to the ECM [40]. Although these mice are viable, they possess a reduced number of pericytes along the capillary bed, and thus represent a tool to investigate the role of pericytes in the adult organism. Analysis of adult pericyte-deficient mice showed that pericytes are important regulators of CNS vascular permeability (discussed in section 3.4.4). Furthermore, pericyte-deficient animals develop brain calcifications at anatomical locations similar to patients suffering from idiopathic basal ganglia calcifications (IBGC) [64]. IBGC is a neurodegenerative disease with an autosomal dominant inheritance pattern. It was recently demonstrated that approximately 25 % of IBGC patients have deleterious mutations in PDGFB or PDGRRB genes [64, 65]. Interestingly, analysis of mice with varying degrees of pericyte deficiency has demonstrated that the extent of brain calcifications correlates inversely with pericyte coverage and BBB defect [64].
Transforming Growth Factor-β
Mouse knockout studies of various genes encoding for components of the TGFβ signaling pathway have demonstrated the importance of the TGFβ signaling pathway in vascular development. TGFβ, together with activins and bone morphogenetic proteins (BMP), form a large superfamily of pleiotropic growth factors that play a role in many different developmental processes. The canonical TGFβ signaling pathway emanates from a ligand binding to a heteromeric receptor complex composed of type 1 (activin receptor-like kinases [ALKs]) and type 2 (e.g. TGFβRII, BMPRII) serine/threonine kinase receptors. Type 1 receptors phosphorylate the transcription factors SMAD2/3, which, upon binding to SMAD4, translocate into nucleus and activate TGFβ target genes. The specific outcome of the cellular response (proliferation, differentiation, migration, etc.) to TGF signaling depends on the repertoire of the type1 receptors expressed by a given cell and the presence of TGFβ co-receptors (e.g. endoglin) (reviewed by ten Dijke and Arthur [66]).
In humans, haploinsufficiency of ligands, receptors, or intracellular effectors causes thoracic aortic aneurysms and dissections (TAADs) [TGFB2, FBN1, TGFBR1, TGFBR2, SMAD3], and hereditary hemorrhagic telangiectasia (HHT) [ENG, ACVRL1, SMAD4]. TAAD patients have aortic dilatations that may develop into aneurysms or aortic dissections, potentially causing sudden aorta rupture and massive bleeding . HHT results in abnormal patterning of the vascular tree, characterized by the formation of arteriovenous anastomoses that predispose HHT patients to frequent hemorrhages. Since TGFβ receptors are expressed both in endothelial cells and mural cells, it has been difficult to assess the precise molecular mechanisms by which TGFβ regulate pericyte recruitment. ALK5 and TGFβRII are expressed by endothelium and pericytes, whereas ALK1 and endoglin are expressed by endothelial cells only. ALK1 induces phosphorylation of SMAD1/5, which promotes angiogenesis, whereas ALK5 signaling in endothelial cells inhibits proliferation acting via the SMAD2/3 complex (reviewed by ten Dijke and Arthur [66]). In addition, TGFβ signaling induces mural cell differentiation, and activation of TGFβ from its latent form requires endothelial–mural cell contact [67]. Thus, endothelial cells and mural are interdependent, with changes in one cell type leading to changes in the other .
The severity of the vascular phenotype of the modified TGFβ signaling pathway is dependent on the developmental stage. TGFβ signaling is not only required for formation of the vascular bed during development but also for vessel maintenance in the adult organism. Most studies that address the role of the TGFβ pathway in mouse vasculature have focused on endothelial-specific constitutive or conditional deletion of TGFβ pathway components (reviewed by Jakobsson and van Meeteren [68]). Generally, genetic ablation of either type 1 (ALKs) or type 2 receptors (TGFβRII, BMPRII) results in hemorrhage, a phenotype often accompanied by vascular smooth muscle defects (reviewed by Jakobsson and van Meeteren [68]). It is often challenging to precisely delineate the role of a single pathway since multiple TGFβ pathways are interdependent and simultaneously active in the cell. For example, ALK1 requires ALK5 kinase expression for its activity [69], and thus genetic ablation of ALK5 affects both pathways. The deletion of genes of the TGFβ/BMP pathways results in a relatively early vascular phenotype and in utero death (around E10) due to the presence of only big trunk vessels such as the dorsal aorta [66]. For example, the conditional of ablation of Bmbr1a, a type 1 receptor that mediates BMP signaling (ALK3), results in embryonic death at E11.5 due to extensive hemorrhage in the trunk [70]. Detailed analysis of the aorta demonstrated reduced mural cell coverage and the poor association between vSMCs and endothelium [70] . Whether this mutation also leads to defective pericyte coverage has not been reported; however, a recent report on ALK5/SMAD2/3 signaling in the endothelium reported poor coverage of mural cells on all caliber vessels, from the aorta to capillaries, which could be due to reduced PDGF-B expression in the endothelium [71]. Increased expression of PDGF-B by thalidomide stimulates mural cell recruitment in endoglin heterozygote animals, an experimental model for HHT [72]. In brain vasculature, the targeted disruption of Smad4, the central intracellular mediator of TGFβ/BMP signaling, also results in poor pericyte coverage along microvessels [35]. In support of this observation, it was demonstrated that TGFβ/BMP and Notch signaling pathways cooperate in the upregulatation of N-cadherin. As discussed above, N-cadherin mediated endothelial/pericyte interaction has been suggested to be important for vessel stabilization [34].
Based on the above studies, it is clear that aberrant TGFβ/BMP signaling in endothelial cells results in poor mural cell coverage. To date, data are not available to explain how pericyte-specific TGFβ signaling affects vessel development or maintenance. However, few studies on the cell-specific elimination of TGFβRII or ALK3 in vSMCs have proven the crucial role of TGFβ/BMP signaling in mural cell proliferation and differentiation along big trunk vessels [73, 74]. Thus, emerging data suggest that TGF signaling in endothelial cells promotes mural cell recruitment, at least partly by inducing PDGF-B expression and also by modulating expression of cell adhesion molecules required for endothelial/pericyte interaction .
Other Pathways
Notch signaling is critical for regulating angiogenesis in both endothelial and mural cells. As noted, Notch signaling is required for mural cell maturation (reviewed by Morrow et al. [75]). In humans, NOTCH3 mutations cause stroke and a syndrome known as cerebral autosomal dominant arteriopathy with subcortical infarcts and leukoencephalopathy (CADASIL), which is associated with dementia. The pathogenic mechanism is not known but may be related to the degeneration of vSMCs caused by accumulated, non-functional Notch3 protein that leads to vessel occlusion and ischemic stroke. Notch3 knockout mice show impaired maturation and reduced coverage of arterial vSMCs [76], but an effect on the pericyte population has not been reported. However, the recent analysis of microvasculature of CADASIL patients has demonstrated the involvement of proper NOTCH3 signaling in pericyte survival [77]. A critical Notch3 ligand on endothelial cells seems to be Jagged-1, which is required for Notch3 expression on vSMCs [78]. It is of note that the endothelial expression of Jagged-1 has been shown to be critical for vSMC differentiation in vivo [79].
The angiopoietin-1 (Ang1)/Tie-2 (Tek) signaling axis has long been suggested to be important for pericyte recruitment. This view is based on the analysis of Angpt1 and Tek knockout embryos that lack mural cell coverage (reviewed by Gaengel et al. [80]). Ang1 is expressed by pericytes and Tie2 is expressed by the endothelium (reviewed Gaengel et al. [80]), yet it remains unclear how the loss of Ang1 or Tie2 results in a defect in pericyte coverage. A recent study has demonstrated that the vascular phenotype observed in Ang1 knockouts is due to abnormal cardiac development [81]. The genetic ablation Ang1 after E13.5 did not alter pericyte recruitment. Consistent with the proposed role of Ang1/Tie2 signaling in regulating vascular stability , absent Angpt1 leads to pathological angiogenesis and tissue fibrosis in the setting of tissue injury or stress [81].
Under in vivo conditions, the heparin-binding epidermal growth factor (HB-EGF)/ErbB signaling pathway in vasculature is important for cardiac development [82]. In addition, in vitro studies have demonstrated the importance of endothelial HB-EGF in mural cell recruitment via ErbB1 and ErbB2 in mural cells [83]. Furthermore, the HB-EGF/Erb pathway has been shown, together with the PDGF-B/PDGFRβ pathway, to regulate pericyte recruitment [84]. Similar to Ang1, HB-EGF seems to play a role in maintaining vascular stability by promoting pericyte survival and proliferation during tissue injury [85].
In tumor vasculature, stromal-derived growth factor-1α (SDF1α) has been shown to promote PDGF-B-dependent pericyte migration via CXCR4 [86, 87]. Interestingly, glioblastoma stem cell recruitment to the endothelium has been shown to be dependent in SDF1α/CXCR4, and their subsequent differentiation into pericytes has been induced by TGFβ [88]. Also, semaphorin 4d-plexin-B1 signaling in endothelial cells was shown to promote pericyte recruitment to tumor vessels in a PDGF-B-dependent manner [89].
Sonic hedgehog (Shh) signaling regulates pericyte recruitment to the choroid plexus [90]. Shh signaling has been also shown to regulate mural cell recruitment during neoangiogenesis [91]. Ephrin-B2 expression in mural cells is needed for proper association between endothelial cells and pericytes [92]. Recently, it was demonstrated that Ephrin-B2 regulates PDGFRβ internalization and thus is important for controlling PDGFRβ signaling activity [93].
3.4 Regulation of Vascular Function
Recent advances using cell purification and culture, genomic analysis and genetic mouse models have identified many different roles for pericytes in development, tissue homeostasis, and disease. These functions include interactions with endothelial cells to regulate angiogenesis, vascular tone and permeability, and interactions with tissue-specific cells to regulate organ development and homeostasis. In addition, pericytes respond to injury and disease by regulating leukocyte trafficking, tissue regeneration, and fibrosis .
3.4.1 Regulation of Angiogenesis
Due to the close proximity of pericytes to endothelial cells, both during development and adulthood, it has long been thought that pericytes play a key role in regulating angiogenesis and vascular stability . Indeed, work with mice with mutations in genes required for pericyte recruitment has demonstrated that these cells play a role in regulating developmental angiogenesis , vascular stabilization, and homeostasis, as well as neoangiogenesis in the adult [80].
During development, many studies have identified the localization of pericytes along the nascent vascular tube and their interactions with endothelial tip cells and stalk cells. Endothelial sprouts have been observed to enter tissues in the absence of pericytes; however, pericytes invade and are recruited to the vascular tube shortly after initial invasion. In fact, pericytes can often be found at the leading edge of the endothelial sprouts interacting with tip cells [18, 94, 95]. It is thought that the endothelial tube plays a role as a migration signal for the pericytes, likely through the secretion of PDGF-BB. Pericytes are recruited either from adjacent vessels or from surrounding mesenchymal precursors.
The timing of pericyte recruitment to the nascent vasculature coincides with vessel stabilization. Indeed, work with mouse mutants that affect pericyte recruitment, such as PDGF-B/PDGFRβ or Ang1/Tie2, have demonstrated that pericytes are critical for vessel stabilization. In these mutants, endothelial cell sprouts are able to enter the developing tissues; however, the vascular tubes often have tortured patterning, increased vessel diameter, and the increased likelihood of hemorrhage [17, 24, 96, 97]. These data suggest that pericytes are not required for the initial invasion of endothelial cells into a tissue, or even the formation of the vascular tube, but they are important for the appropriate patterning of the vasculature as well as stabilizing the vascular tube. Corroborating these data are in vitro co-culture models that have demonstrated that pericytes can supress endothelial cell growth and migration [98, 99]. Furthermore, pericyte recruitment to the nascent vessels has been implicated in resistance to regression of the newly formed sprouts [100].
Pericytes have also been implicated in neoangiogenesis in adults in response to alterations in oxygen levels, injury, or disease. In the initial phase of angiogenic processes, pericyte cell bodies bulge and increase in volume, their processes shorten, and they proliferate [101]. In addition, they increase their expression of MMPs, such as MMP-9, which corresponds to the disruption of the basement membrane required for the migration of new angiogenic sprouts [102]. This process may be guided by the same signals that regulate developmental angiogenesis . Vascular endothelial growth factor (VEGF) production has been shown to induce proliferation and migration of pericytes during hypoxia via an indirect mechanism through the production of nitric oxide (NO) by the endothelium [103, 104]. In addition, VEGF can be produced by the pericytes to direct this process themselves [105] .
Pericytes have also been implicated as important regulators of tumor angiogenesis and thus are potential targets in antitumor therapy [106]. Studies have identified pericytes on blood vessels along the leading edge of tumor growth with incomplete pericyte coverage implicated in vascular abnormalities, and increased vascular permeability has been observed in different tumors [107–109]. Anti-VEGF treatment, which targets the endothelium, has been shown to remove tumor vessels while sparing pericytes [110]. Alternatively, it also leads to vessel normalization with increased pericyte coverage [111, 112]. Although targeting of pericytes through inhibition of PDGFRβ showed no loss of tumor vasculature [113, 114], inhibition of PDGFRβ combined with anti-endothelial therapy has provided synergistic success in reducing tumor vasculature and size in multiple tumor models [115]. Therefore, targeting of pericytes to decrease tumor vasculature is an active area of research .
3.4.2 Regulation of Blood Flow
Almost 100 years ago, Krogh and co-workers first reported remote vasodilation of capillaries in response to neural stimuli (reviewed by Bagher and Segal [116]). Focal capillary stimulation has also been shown to affect local blood flow. Specifically, fluctuations in capillary blood flow have been explained by conduction of vasomotor responses by electrical signals via endothelium that leads to relaxation of vSMCs at distinct locations within the resistance network (reviewed by Bagher and Segal [116]). Pericytes, similarly to vSMCs, express contractile proteins and have the capacity to contract in response to vasodilative and vasoconstrictive agents in vitro (reviewed by Rucker et al. [117, 118]). Although the regulation of blood flow by pericytes seems plausible, (in fact, it was the first function suggested for pericytes), it has been difficult to demonstrate in vivo. Changes in the diameter of arteries and arterioles (i.e. vessels that have vSMC coverage) are thought to regulate blood flow in response to different metabolic needs. For example, in the brain, the regulation of functional hyperemia (i.e. blood flow increases in response to increased local neuronal activity) is initiated by neurotransmitters released by neurons, and occurs at the level of the arterioles (reviewed by Attwell et al. [119]). Based on the demonstration of pericyte-mediated constriction of vessels in ex vivo cerebellar slices and retina preparations [120, 121], it seems plausible that in vivo pericyte-mediation is possible at the level of capillaries in response to neuronal-stimuli. Although pericytes were shown to constrict in vivo, a role for pericytes in neurovascular coupling was difficult to identify [122]. However, a recent study reported that vasodilation and blood flow changes in the somatosensory cortex were initiated in capillaries after whisker pad stimulation [121]. Vasodilation of capillaries occurred, on average, 1.4 s before vasodilation was seen in the arterioles, thus implicating that vasodilation in capillaries is not a passive response to pressure changes occurring at the level of arterioles [121]. In addition, in pathological conditions, pericyte-mediated contraction could potentially disturb the flow. Pericyte-mediated contraction in response to ischemia was shown to constrict capillaries in vivo, suggesting that pericytes contribute to the ‘no-reflow’ phenomenon after cerebral ischemia [123]. Neural stem cell progenitor proliferation in the neurogenic niche in the subventricular zone is accompanied by an increase in local capillary bed blood flow. It was recently shown that specialized subventricular zone astrocytes (B cells) and neural progenitors regulate flow in the capillary bed by purinergic receptor activation on pericytes [124].
The kidney is another organ where pericytes are purported to regulate capillary blood flow. The renal medulla is vascularized by vasa recta capillaries, and blood flow in these capillaries requires tight control to facilitate the concentration of urine and avoid severe ischemia in the medulla. Similar to brain microvasculature, regulation of renal medullary blood flow may occur at the level of the capillary bed (vasa recta), independent of arterial perfusion pressure (reviewed by Kennedy-Lydon et al. [118]).
3.4.3 Regulation of Leukocyte Trafficking
For leukocytes to enter tissue, a tightly orchestrated series of events takes place on blood vessel walls. The leukocyte adhesion cascade involves rolling, activation, adhesion strengthening, intraluminal crawling, firm adhesion, and paracellular and transcellular migration. Leukocyte entry is crucial for immune surveillance and the control of tissue inflammation . Although there is extensive knowledge on the cellular and molecular mechanisms by which leukocytes cross the endothelium , relatively little attention has been paid to the role of pericytes in these processes.
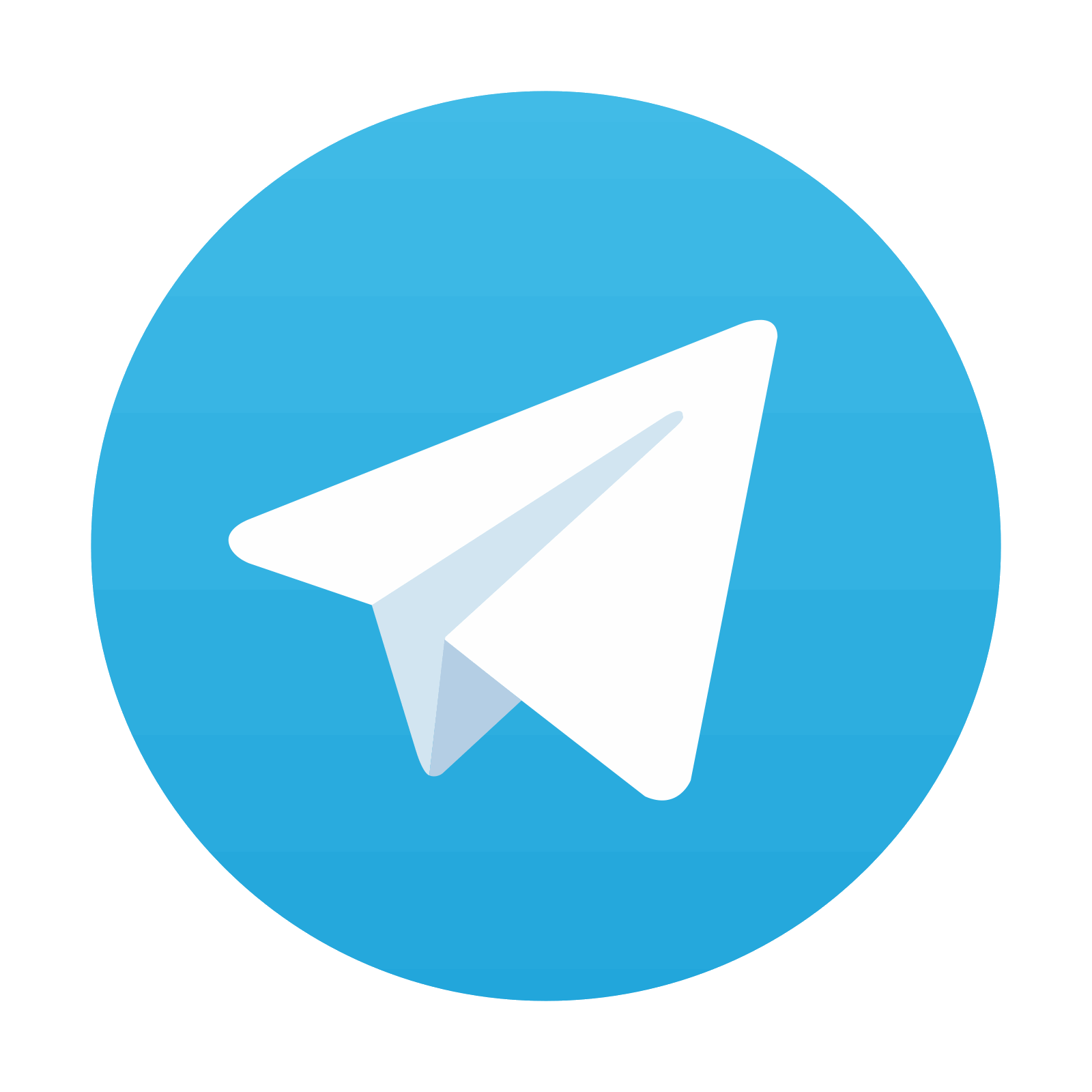
Stay updated, free articles. Join our Telegram channel
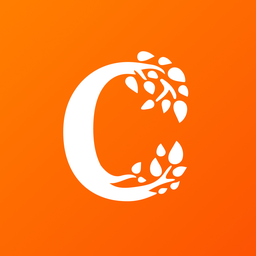
Full access? Get Clinical Tree
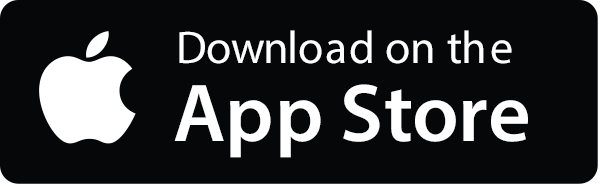
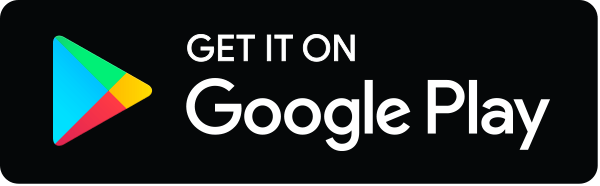