Fig. 14.1
Domain structure for hypoxia-inducible factors (HIFs), prolyl hydroxylase domain proteins (PHDs), and factor-inhibiting HIF (FIH). a Schematic diagram of the HIF-1α, HIF-2α, and HIF-1α proteins and their functional domains. HIFs contain two Per-ARNT-Sim (PAS) domains (PAS-A and PAS-B), which are required for heterodimerization and DNA binding. HIF-1α and HIF-2α have an N- and C-terminal transactivation domain (N-TAD and C-TAD), which are linked by an inhibitory domain (ID). Two prolyl hydroxylation sites (prolines 402 and 564 in HIF-1a and prolines 405 and 531 in HIF-2α) are located within the oxygen-dependent degradation domain (ODD) and an asparaginyl hydroxylation site (asparagine 803 in HIF-1α and 847 in HIF-2α within the C-terminal transactivation domain. b Schematic diagram of PHD and FIH proteins and their functional domains. All hydroxylases contain a conserved catalytic dioxygenase domain at their C-terminal ends. PHD1 and PHD2 have a nuclear localization signal (NLS) and PHD2 also contains a nuclear export signal (NES). Additionally, PHD2 has a zink-finger domain (ZnF)
Thus, when considering the oxygen-related responses on the cellular level, it is interesting to note in this context that the vasculature itself does not only serve as a well-defined oxygen distribution network but is also highly responsive to differences in oxygen tension itself. It has been reported that endothelial cells in the growing vasculature are functionally distinct and exhibit a specific molecular signature [22–25]. These findings are particularly interesting in light of the notion that a growing vessel is exposed to an oxygen gradient with the lowest oxygen level at the forefront of the growing sprout, generally known as the tip cells . In comparison to that, the neighboring stalk cell experiences higher oxygen levels, exemplifying how oxygen signaling contributes to determine the endothelial cell fate and thereby impacts on angiogenic processes. Mechanistically, initiation of the oxygen-sensing machinery encompasses a very complex and broad response; it is generally known that the HIF system alone already regulates the expression of over 100 target genes [26]. Multiple pro- and antiangiogenic players are induced, providing compelling evidence that hypoxia is one of the key environmental cues that trigger blood vessel growth under physiological and pathophysiological conditions [27]. Physiological angiogenesis is an accurately controlled process where pro- and antiangiogenic factors need to be strictly balanced to assure that the interaction between different cell types occurs in an orderly fashion. Once this equilibrium is disturbed, the excessive production of specific angiogenic players will lead to abnormal vessel growth, with long-lasting consequences, as evinced by the chaotic structure of tumor vessels [28]. Gaining further mechanistic insights from physiological angiogenic processes such as embryonic development , reproduction, or wound healing might help to uncover to what extent these mechanisms are coopted and exploited under pathophysiological conditions. Having outlined the general importance of mammalian oxygen sensing, in the following section we will proceed to provide a detailed description regarding the components of the oxygen-sensing machinery.
14.2 The Role of Hypoxia-Inducible Factors (HIFs) in Oxygen Signaling
In the last two decades a large number of studies have been centered on the HIF system and its implication in the role of oxygen in health and disease. This marked interest was captivated by the identification of the HIF in 1995 (Wang and contributors [29]), and the characterization of the PHDs as regulators of HIF stability (Epstein and co-workers [15], and Bruick and McKnight [14]) at the beginning of the twenty-first century. Initially, mechanistic studies regarding the strong induction of erythropoietin under hypoxic conditions led to the discovery of an hypoxic responsive element (HRE; 5ʹ-RCGTC-3ʹ) in the 3ʹ enhancer of the erythropoietin gene [30]. This consensus sequence is very well-conserved between different species, pointing towards the evolutionary importance of oxygen sensing . Subsequently, a protein binding to this specific DNA sequence could be identified as the HIF, regulating the hypoxic-dependent erythropoietin expression [29]. HIF is composed of the hypoxia-inducible α-subunit (HIF-1α) and a constitutively expressed β-subunit (HIF-1β) [29]. HIF-1β was previously identified as a binding partner of the aryl hydrocarbon receptor, thus being referred to as aryl hydrocarbon nuclear translocator (ARNT) [31]. Succeeding the cloning of HIF-1α, a closely related protein (HIF-2α), sharing 48 % of amino acid identity, was discovered in 1997 [32–35]. To date, it is generally accepted that there are three HIF-α proteins. HIF-1α and HIF-2α are positively associated with HIF target gene expression, whereas the inhibitory Per-ARNT-Sim (PAS) domain (IPAS), an HIF-3α isoform, is reported to serve as a negative regulator of HIF signaling [36, 37]. HIF-1α and β contain a basic helix-loop-helix (bHLH) and PAS domain in the N-terminal half of the protein [29]. These domains are important for its dimerization, and the downstream basic region enables specific DNA binding to the HRE sequence [29, 38]. Subsequently, a C- and N-terminal transactivation domain could be located within the C-terminal half of the HIF-α subunit, specifying the transcriptional activity of HIF [39–41] (Fig. 14.1a). Of note, an important transcriptional regulation is accomplished by FIH-dependent asparaginyl hydroxylation within the C-terminal transactivation domain, sterically inhibiting the recruitment of transcriptional coactivators such as cyclic adenosine monophosphate (cAMP) response element-binding protein CBP/p300 [17, 35, 42] (Fig. 14.2).

Fig. 14.2
Regulation of hypoxia-inducible factor (HIF) degradation and transcriptional activity by prolyl hydroxylase domain proteins (PHDs) and factor-inhibiting HIF (FIH), respectively. In the presence of divalent iron (Fe 2+ ), 2-oxoglutarate, and oxygen, PHDs catalyze the hydroxylation of HIF at two prolyl residues, which leads to their recognition by the E3 ubiquitin ligase von Hippel Lindau protein (pVHL), and consequently to ubiquitination and proteasomal degradation of HIF. FIH hydroxylates HIF at an asparagine residue, which hinders the interaction between FIH and its coactivators CBP and p300, and thus leads to transcriptional inhibition. If oxygen levels drop, PHDs and FIH become partly inactive and HIF-α is stabilized, translocates to the nucleus where it heterodimerizes with nuclear HIF-1α heterodimer, and binds with its coactivators to the hypoxic responsive element (HRE) of target genes, initiating target gene expression
There are a number of post-translational regulations of the HIF protein, such as hydroxylation, phosphorylation, acetylation, and sumoylation [14, 15, 42–49]. Hydroxylation within the oxygen-dependent degradation domain (ODD) by PHDs is an important determinant of HIF protein stability. Hydroxyproline HIF is recognized by the von Hippel Lindau protein (pVHL), which is part of an E3 ubiquitin ligase complex subsequently leading to ubiquitination and proteasomal degradation of HIF [50–52] (Fig. 14.2).
As aforementioned, HIF-1β messenger RNA (mRNA) and protein are constitutively expressed; however, in contrast, the HIF-1α protein is very unstable and its availability strongly depends on the oxygen tension [53]. In this context, it should be noticed that the transcriptional processes regarding HIF-1α are not strictly regulated by oxygen [54]. Importantly though, the rate of HIF turnover functions as an oxygen-dependent checkpoint. HIF is rapidly degraded under normoxic conditions (t½ = 5 min) and accumulates when oxygen levels drop, since degradation via the proteasomal pathway is hampered [55]. This allows HIF to translocate to the nucleus, where it binds as a heterodimer consistent of HIF-1α and HIF-1β to the consensus sequence within the hypoxic response element of target genes [29, 31]. Recruitment of coactivators enables the initiation of the transcriptional complex, resulting in the expression of a number of target genes [56, 57] (Fig. 14.2)
Interestingly HIF-1α and HIF-2α possess overlapping and nonredundant functions [58, 59]. Spatial-temporal differences in the expression exist [60]. Whereas HIF-1α is almost ubiquitously expressed, the abundance of HIF-2α is more restricted to specific cell types, among which are endothelial cells and the carotid body [35, 61, 62]. HIF-2α was also shown to already accumulate under higher oxygen tension, supporting the idea that each isoform might serve a specific function [60]. Since the availability of HIF protein is robustly interconnected with the oxygen tension, in the following section we will focus on the PHD-dependent regulation of HIF turnover.
14.3 The Function of Prolyl Hydroxylase Domain Proteins (PHDs) and Factor-Inhibiting HIF as Oxygen Sensors
Oxygen-dependent HIF regulation takes place at post-transcriptional level. One of the main factors involved in the turnover of HIF proteins are the PHDs (Fig. 14.1b). PHDs belong to a family of nonheme iron- and 2-oxoglutarate-dependent enzymes [14, 15, 51, 52]. The hydroxylation reaction encompasses oxygen and 2-oxoglutarate as cosubstrates, and ferrous iron and ascorbate as cofactors. During the enzymatic reaction, one oxygen atom is utilized to form HIF hydroxyproline, while the other is required for the decarboxylation of 2-oxoglutarate which results in the formation of succinate and CO2. Fe2+ is bound to the active site of the PHD protein, and oxidized during the enzymatic reaction. Ascorbate is needed to reduce the iron during the reaction cycles; however, the consumption does not occur stoichiometrically, therefore leaving room for further investigation [63] .
Hydroxylation occurs on Pro402 and Pro564 within the LXXLAP sequence of the ODD in human HIF-1α, and Pro405 and Pro530 in human HIF-2α [64, 65]. Corresponding to their relative proximity to N- and C-termini, these residues are denoted N-ODD or C-ODD, despite their location within the C-terminal halves of the protein. There are three PHD proteins (PHD1–3), and hydroxylation activity on proline 564 is higher for all PHDs; notably, PHD3 is principally inactive at proline 402 [66, 67]. Interestingly, PHDs also differ in their selectivity of HIF-1α versus HIF-2α hydroxylation. While PHD2 exhibits a higher hydroxylation activity on HIF-1α than on HIF-2α, PHD1 and PHD3 preferentially hydroxylate HIF-2α in comparison to HIF-1α [66, 67]. Additionally, FIH was shown to more potently hydroxylate HIF-1, thus contributing to HIF-1- and HIF-2-specific functions [68].
PHD2 is known to be the main HIF regulator under normoxic conditions [69]. Nevertheless there is a growing body of evidence that, dependent on their availability, the relevance of the respective PHD protein might shift. Their functionality might be designated by cell-type specificity, HIF isoform availability, and environmental cues. For instance, PHD2 and, more prominently, PHD3 are induced in an HIF-dependent manner in hypoxia. HIF binding to the HRE could be manifested for both proteins [70, 71]. Interestingly though, HIF-1α and HIF-2α differentially induce PHD2 and PHD3. Whereas HIF-1α induces both PHD2 and PHD3, HIF-2α only enhances PHD3 expression [72, 73].
It is noteworthy that PHDs themselves suppress HIF transcriptional activity, further adding to the complexity of the system [74, 75]. When associating with inhibitor of growth family, member 4, (ING4), a tumor suppressor gene, PHD2 was shown to impede HIF-dependent expression of angiogenic cytokines via transcriptional repression [76]. Thus, in addition to the well-described oxygen-dependent control of HIF and PHDs, other mechanisms are involved in a regulatory function of the HIF response, among them, for instance, receptor-mediated pathways [77]. The knowledge regarding oxygen-independent HIF and PHD regulation will be briefly summarized in the following section .
14.4 Oxygen-Independent HIF and PHD Regulation
There is evidence that HIF target gene expression is enhanced by two well-characterized signaling pathways, the PI3K-AKT-FRAP and Ras-MEK-MAPK cascade [77–79]. First evidence of HIF-induced target gene expression through such a mechanism stems from studies on insulin and insulin-like growth factor (IGF)-1 [80]. Subsequently, an increasing number of growth factors, cytokines, and circulating factors such as platelet-derived growth factor (PDGF), epidermal growth factor (EGF), fibroblast growth factor (FGF)-2, IGF-2, transforming growth factor (TGF)-1β, hepatocyte growth factor (HGF), tumor necrosis factor (TNF)-α, interleukin (IL)-1β, angiotensin-2, and thrombin were found to enhance HIF1α-dependent target gene expression [81–87]. In addition, oncogenes (HER2/neu, Ras, v-Src) and mutations in the tumor suppressor PTEN modulate HIF activity [88–91]. Overall, integration of growth factor signaling occurs at the transcriptional as well as post-transcriptional level. For instance, phosphorylation by mitogen-activated protein kinases (MAPK) enhances HIF transcriptional activity, possibly via derepression of the HIF inhibitory domain [45]. This phosphorylation might hamper recognitions and subsequent binding of FIH, consequently enhancing HIF transcriptional activation. On the post-transcriptional level, induction of the PI3K-AKT-FKBP12 rapamycin-associated protein (FRAP)/mammalian target of rapamycin (mTOR) pathway by various cytokines enhances HIF mRNA translation [92]. Given the complexity of growth factor signaling, activation of a kinase cascade, and the subsequent HIF response is profoundly dependent on the cellular context.
Nitric oxide (NO) is known to be a mediator of angiogenesis. Due to its implication in serving a prosurvival and proangiogenic function and its involvement in vasodilation [93], the impact of NO on the PHD/HIF signaling axis will be discussed. NO elicits opposing effects on HIF stability, depending on the oxygen state of the cell. Under normoxia, NO exhibits an inhibitory function on PHD activity, possibly by chelating Fe(II) consequently reducing HIF turnover [94]. Mechanistically, NO inactivates cytochrome c oxidase, impedes ROS production, and causes a redistribution of the available oxygen to the cytosol. However, since oxygen is not a limiting factor under normoxic conditions, this redistribution remains without consequences. However, under hypoxic conditions, these effects gain in importance and lead to activation of PHDs and concomitantly diminished HIF abundance [95]. However, HIF modulation by NO seems to be more complex as it has been shown that the specific experimental conditions critically influence the impact on the HIF signaling axis [96]. Finally, metabolites are also associated with an HIF modulatory function. HIF stability is enhanced by pyruvate, lactate, and oxalacetate, presumably through inhibiting of PHD function [97–99].
The regulation of PHD1 and PHD3 protein stability is again linked to an hypoxic response. The RING finger proteins seven in absentia (Drosophila) homologs 1/2 (SIAH 1/2) were shown to target PHD1 and, most efficiently, PHD3 for polyubiquitination and proteasomal degradation, thereby accomplishing, at least to some extent, PHD1/3 protein destruction. Hypoxia induces SIAH 2 accumulation, most likely in an HIF-independent manner, and thus SIAH 2 controls hypoxic PHD3 abundance in a negative feedback regulatory loop [100]. PHD2 protein destruction is positively regulated by FKBP38, a peptidyl prolyl cis/trans isomerase. Silencing of FKBP38 does not affect PHD2 mRNA but enhances its protein stability [101].
Having obtained a comprehensive understanding of oxygen sensing on a molecular level in the last two sections, we will zoom out and discuss in the next section the functional relevance of these processes during physiological blood vessel remodeling.
14.5 Role of Oxygen Signaling on Physiological and Pathophysiological Angiogenesis
In a very simplified view, the establishment of a vascular system can be subdivided into two processes, vasculogenesis, which refers to the de novo formation of blood vessels from endothelial precursor cells during embryonic development, and angiogenesis, which specifies the process of new blood vessel emergence from pre-existing ones [102, 103] . In the embryo, angiogenesis occurs after the primary capillary plexus has been formed. In the adult, angiogenesis is a relatively scarce event, almost exclusively constrained to the ovarian cycle or remodeling processes after injury such as wound healing. A number of very well coordinated events guarantee the establishment of new blood vessels from an existing vascular network. This involves destabilization of vessels by loosening the attachment of pericytes, the subsequent digestion of the basement membrane as well as the extracellular matrix surrounding the blood vessels and its remodeling, and new matrix synthesis. It further includes the ensuing activation of endothelial cell proliferation and migration stimulated by the newly formed matrix until endothelial cells arrest and form tube-like structures. They are successively covered by pericytes to ensure the establishment of a tight network, allowing an efficient blood flow. During this process, endothelial cells are exposed to gradients of different pro- and antiangiogenic factors as well as gradients of oxygen levels [28] .
Exemplified by vascular endothelial growth factor (VEGF), one of the most characterized angiogenic factors, it becomes apparent how oxygen sensing is intertwined with the angiogenic process. VEGF abundance is regulated by oxygen tension, as low local oxygen abundance leads to an HIF-driven VEGF expression [104, 105] .
This soluble factor exerts a number of different functions during angiogenesis. To name a few, it participates in the destabilization of vessels as it impairs the physical barrier formed by tight junctions between endothelial cells [106, 107]. Furthermore it contributes to endothelial proliferation and migration via binding to VEGF receptor-2 (VEGFR2) expressed at the forefront of the endothelial sprouts, the tip cells . As aforementioned, the cells that trail behind, the stalk cells, differ in their gene expression profile. The tip versus stalk cell phenotype is among others specified by VEGF-mediated activation of VEGFR2 that leads to upregulation of the Notch ligand Dll4 in tip cells and subsequently enhanced Notch signaling and downregulation of VEGFR2 in adjacent stalk cell [108] (Fig. 14.3). However, in addition to regulating the production of angiogenic factors, and thus subsequently triggering the activation of a specific signaling cascade, oxygen levels might also more directly influence the endothelial cell fate .

Fig. 14.3
Prolyl hydroxylase domain protein-2 (PHD2) is an important determinant of the switch from a motile tip cell to a quiescent phalanx cell phenotype. Outgrowth of vessel sprouts is triggered by proangiogenic growth factors such as vascular endothelial growth factor (VEGF). Cells exposed to the highest concentration of proangiogenic factors are selected to become a tip cell with filopodia invading their surroundings. Proliferating stalk cells that trail behind elongate the sprout. While the growing vessel strikes out alongside a gradient of increasing proangiogenic factors, it encounters decreasing concentrations of oxygen, which concomitantly inhibit PHD and factor-inhibiting hypoxia-inducible factor (FIH) activity (1 and 2). Two vessel sprouts connect via tip cell fusion in highly hypoxic/anoxic regions. Inactivation of PHD2 function by oxygen scarcity (3) favors the switch from a motile tip cell phenotype to a quiescent phalanx phenotype (4). Thus, perfusion is established and PHDs are reactivated (5); however, the absence of proangiogenic players (following tissue reoxygenation) guarantees a quiescent phenotype will be maintained
In this context, it is of interest that Dll4 is regulated in an HIF-dependent manner in response to hypoxia [109], and that Notch intracellular domain (NICD) activity is negatively controlled via FIH [110]. Moreover, FIH exhibits even higher affinity for Notch ICD than for HIF [111]. Hydroxylation of Notch ICD at specific asparagine residues leads to negative regulation of its transcriptional activity. In addition, Notch ICD was shown to positively regulate HIF target gene expression, most likely by competing with HIF for FIH hydroxylation [112]. Thus, the Notch–FIH–HIF signaling interplay might be an interesting determinant for the endothelial cell fate.
It has already been shown that reduced activity of the HIF prolyl hydroxylase PHD2 in endothelial cells evokes a quiescent, ‘phalanx’ phenotype that involves a closer alignment of cells and thus fosters a more mature and better-perfused vascular network [113]. Growing vessels are exposed to oxygen gradients, with higher oxygen concentrations at the side of the stalk cells and subsequently decreasing oxygen levels towards the tip cells . However, the gradient of angiogenic factors is opposite to the oxygen distribution as outgrowth of vessel sprouts is triggered by proangiogenic growth factors such as VEGF produced by the hypoxic tissue. Tip cell selection occurs where cells are exposed to the highest concentration of proangiogenic factors and proliferating stalk cells elongate the sprout alongside increasing VEGF and decreasing oxygen concentration. At the point where two vessel branches connect, PHD activity is, at least in part, inhibited due to oxygen deprivation. This favors the switch from a motile tip cell phenotype to a quiescent phalanx phenotype (Fig. 14.3) .
FIH was shown to exhibit lower affinity for oxygen than PHD2 [66, 114], thus being operational under lower oxygen concentration and inactive at nearly anoxic conditions. Therefore, and with respect to the HIF–FIH–Notch interplay, it will be of particular interest for future studies to dissect the physiological relevance of FIH in endothelial cell types that experience severely hypoxic/anoxic conditions such as the migratory tip cells, and to further define whether the endothelial cell fate (tip vs. stalk cell) is interconnected with FIH functionality .
Evidence from genetic studies has formed the general opinion that HIF-1α and HIF-2α hold nonredundant functions in angiogenesis. Specific deletion of HIF-1 in endothelial cells results in a malfunctioning vascular network under hypoxic conditions such as in tumors [115] . HIF-1 deficiency attenuated hypoxia-induced target gene expression, and this disturbed hypoxic response is not compensated by HIF-2α underlining their distinct roles. Functionally, the genetic loss of HIF-1α hampers endothelial proliferation and migration, and interferes in an autocrine VEGF/VEGFR2 loop since the expression of both is under the control of HIF-1α. While the HIF-1α isoform is ubiquitously expressed, HIF-2α expression is restricted to a subset of cell types, among which are endothelial cells. In particular, HIF-2α is highly expressed in endothelial cells during development, pointing towards a functional relevance in this cell type [32]. Endothelial-specific deletion of HIF-2α disrupts vessel functionality, resulting in smaller and more hypoxic tumors, or impaired and aberrant revascularization of ischemic tissues. Mechanistically, loss of HIF-2α in endothelial cells impairs cell adhesion and thus impacts on vessel functionality [116] .
In line with a role of this isoform in vessel integrity, in a different study HIF-2α deletion in endothelial cells caused aberrant tumor vessel growth via reduced expression of ephrin A1 [117]. When considering HIF-2α as an important player in vessel normalization, remodeling, and maturation, it should also be pointed out that endothelial NO sunthase (eNOS) as well as the junctional protein vascular endothelial (VE)-cadherin, are under the specific control of HIF-2α and not HIF-1α [118, 119]. Interestingly, the metastatic spread of tumor cells seems to be differentially governed by HIF-1α and HIF-2α. While endothelial-specific deletion of HIF-1α diminishes lung metastasis, HIF-2α loss enhances the metastatic spread. This effect can be attributed to HIF isoform-specific regulation of NO homeostasis, with HIF-1α loss resulting in reduced NO release and HIF-2α deletion enhancing it. The contrasting HIF-1α/HIF-2α-dependent regulation of NO release results in opposite effects on endothelial tumor cell transmigration. An HIF-1α-deficient endothelium enhances, and an endothelium with disrupted HIF-2α expression inhibits, the transmigration of tumor cells [120] .
As outlined above, genetic deletion of PHD2 results in tumor vessel normalization, namely improved endothelial lining, barrier, and stability, carried out through an HIF-2α-driven response. These structural changes warrant a better vessel perfusion and thus tumor oxygenation. As a consequence, tumor cell intravasation and metastasis are reduced [113]. To date, there are no reports for a causal link between endothelial-specific PHD1 and/or PHD3 deletion and vessel malfunction. Overall, inhibition of PHDs in endothelial cells promises a beneficial outcome for cancer treatment. In which way the oxygen-sensing machinery might be exploited for future therapeutic approaches will be the focus of the following section.
Given the fact that oxygen is the main substrate of aerobic organism, it follows that PHDs, and thus oxygen sensing, are closely intertwined with cellular metabolic responses [121–123]. In this respect, a lot of effort has recently been undertaken to understand in which way a metabolic switch can be a cause rather than a consequence of a phenotypic change. A rather new observation suggests the involvement of endothelial cell metabolism in vessel sprouting [124]. In this study, the authors showed that the hypoxia-driven release of the proangiogenic factors VEGF and FGF2 trigger 6-phosphofructo-2-kinase/fructose-2,6-bisphosphatase 3 (PFKFB3) mediated glycolysis and favor the tip cell phenotype . Strikingly, PFKFB3 signaling even predominates Notch signaling, as evinced by overexpression of both PFKFB3 and NICD in a mosaic-sprouting assay, resulting in a preferred tip cell positioning of these cells. Interestingly, PFKFB3 silencing or genetic deletion does not only impair the tip cell behavior but also affects the stalk cell function by evoking a hypoproliferative stalk cell phenotype [124]. Thus, given the oxygen control of cellular metabolism, these findings uncover an additional control mechanism of vascular sprouting .
14.6 Oxygen-Sensing Pathways as Future Therapeutic Targets
Due to the interrelation between disturbed oxygen homeostasis and pathophysiological conditions such as stroke or cancer, it stands to reason that oxygen signaling pathways are coopted to facilitate disease progression. Interfering in these signaling pathways holds the promise of new and beneficial treatment options. First, evidence for potentially improved curing prospects when targeting oxygen-sensing enzymes stems from drug-mediated inhibition of PHDs, which stimulates angiogenesis and generates a more mature vascular network [125, 126]. However, genetic studies allow a more profound understanding of how interfering in the function of one specific oxygen-sensing enzyme might alter disease progression.
Of utmost importance for cancer therapeutics are the findings that chemotherapeutic treatments in combination with genetic deletion of PHD2 leads to a beneficial outcome [127]—on the one hand, because tumor growth and metastasis are reduced and, on the other hand, as chemotherapeutic side effects on healthy organs are diminished (Fig. 14.4). Endothelial-specific PHD2 deletion alone was previously shown to normalize the tumor vasculature and reduce metastatic tumor cell spread, but without influencing the primary tumor growth [113]. Of note, the combinatory approach of disrupted PHD2 expression and the use of suboptimal doses of chemotherapeutics results in a self-reinforcing antitumor and antimetastatic effect, holding the promise for improved chemotherapeutic regimens. Normalization of the tortuous tumor vasculature through inhibition of PHD2 function allows a better distribution of chemotherapeutics within the cancerous tissue, thus leading to more effective elimination of malignant cells. Importantly, the presence or absence of PHD2 in cancer cells does not alter the response underlining the translational potential of these findings as drug-mediated inhibition would likewise target different cell types at the same time [127]. Nevertheless, medication-mediated intervention on the FIH/PHD/HIF axis warrants caution. One prerequisite is the development of specific inhibitors for the oxygen-sensing enzymes. FIH and the respective PHD isoforms have been shown to differentially regulate HIF-1α and HIF-2α (see Sect. 3). Given the nonoverlapping and even opposing functions of HIF-1α and HIF-2α in endothelial cells under pathophysiological conditions (e.g. their isoform-specific regulation of metastatic tumor cell spread [120], inhibitors with unique selectivity need to be applied.

Fig. 14.4
Prolyl hydroxylase domain protein-2 (PHD2) inhibition in combination with chemotherapy is beneficial for cancer treatment. Endothelial deletion of PHD2 normalizes chaotic tumor vessels, decreases tumor hypoxia (brown areas), and inhibits metastatic tumor cell spread, but does not affect primary tumor growth (box with blue background). Suboptimal doses of chemotherapy do not inhibit primary tumor growth but result in cytotoxic side effects, which compromise organ functions (box with yellow background). A combinatory approach of suboptimal doses of chemotherapeutics and inhibition of PHD2 further diminishes metastatic tumor cell dissemination but also decreases primary tumor growth as better perfused vessels allow chemotherapeutics to reach their target cells and execute their functions. Importantly, PHD2 deletion protects healthy organs from adverse side effects (box with half blue, half yellow background) because of detoxifying responses in normal cells
This might not only bypass unwanted side effects engendered by induction of unexpected HIF isoform-mediated functions but might also circumvent effects evoked by interference in HIF-independent PHD- or FIH-specific signaling pathways as there is increasing evidence for HIF-independent interaction partners of oxygen-sensing enzymes that might critically alter the response [128].
However given the exceeding importance of oxygen signaling in angiogenic processes and their relevance in therapy induced responses, pharmacological exploitation of oxygen-sensing pathways might serve as a goldmine for future drug discovery .
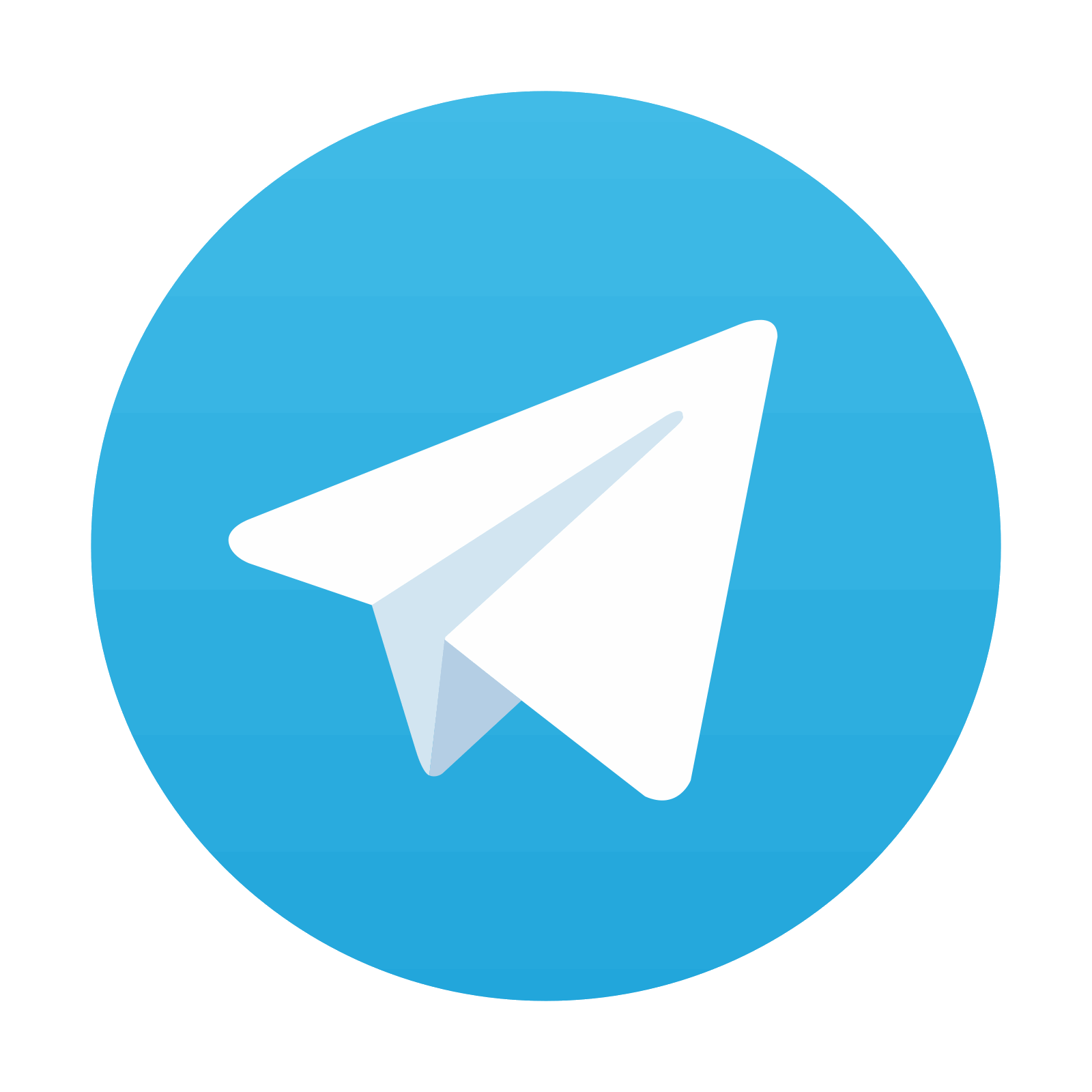
Stay updated, free articles. Join our Telegram channel
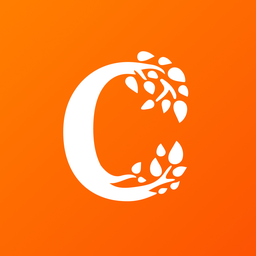
Full access? Get Clinical Tree
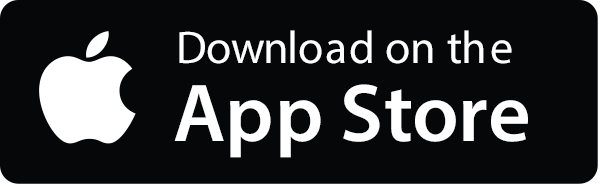
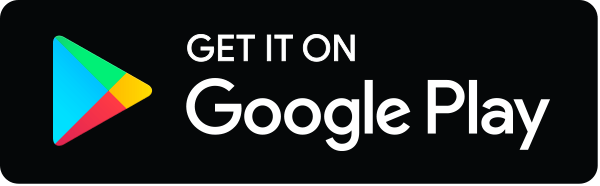