Fig. 18.1
The primary antioxidant enzymes and their function
Table 18.1
Different types of reactive oxygen species (ROS)
Radicals |
Non-radicals | ||
---|---|---|---|
Hydroxyl |
OH− |
Hypochloric acid |
HOCl |
Superoxide |
O2 −● |
Hydrogen peroxide |
H2O2 |
Thyl |
RS−● |
Lipid peroxide |
LOOH |
Peroxyl |
RO2 ● |
Ozone |
O3 |
Lipid peroxyl |
LOO● |
Singlet oxygen |
−1O2 |
All cellular components including nucleic acids, lipids, and proteins are potentially OS targets as ROS are present in almost all tissues [31]. Free radicals predominantly attack the closest stable molecule altering that molecule and its characteristics. ROS can be involved in a cascade of reactions which can damage a wide variety of biomolecules [32, 33]. OS can lead to oxidation of membrane lipids, DNA-related carbohydrates and amino acids, thereby affecting cell function and endangering cell survival [9, 29, 34]. The reproductive system is specifically vulnerable to oxidative damage as the plasma membranes of spermatozoa and testicular tissue are particularly rich in polyunsaturated fatty acids (PUFA) [35, 36]. Damaged sperm plasma membranes result in reduced sperm motility and ability to fertilize the oocyte. DNA damage has also been observed to be a common effect of OS. Damage, such as single and double strand breaks to the spermatozoon’s DNA affects the embryo’s paternal genetic contribution, and thus OS can have a telling influence on fertilization and development of the embryo [15]. Infertile patients presenting with normozoospermia, who are categorized as idiopathic infertile patients, generally present with higher levels of ROS and lower levels of antioxidants. This observation emphasizes the link between idiopathic infertility and OS and the need for more knowledge with regard to this subject [37, 38]. However, it is important to take note that a delicate balance exists between ROS and antioxidants, especially within the reproductive system. This is considered necessary for processes such as spermatogenesis to occur routinely, and it is only when this homeostasis is disturbed that malfunction occurs. An ideal example of this concept is hydrogen peroxide, which plays an integral role in tyrosine phosphorylation and the acrosome reaction. Hydrogen peroxide is therefore essential for proper binding of the spermatozoa to the zona pellucida [9, 29, 34]. Catalase, the antioxidant reducing hydrogen peroxide into water and oxygen, has also been indicated to be of importance for the preservation of sperm motility. Thus, the maintenance of a proper oxidative status in the reproductive system is essential to fertility and disturbing this harmony promotes subfertility [15].
Sources of ROS
While noted in the previous section that ROS are produced as a natural by-product of ATP generation, certain lifestyle choices and environmental exposures can also elicit excess ROS production and lead to OS. It is therefore important to identify possible sources of OS to improve oxidative infertility treatment/management. The following exposures have been linked to OS (as shown in Fig. 18.2):

Fig. 18.2
Endogenous and exogenous sources of reactive oxygen species (ROS) and reactive nitrogen species (RNS) that can lead to oxidative stress (OS) if the balance with antioxidants is disturbed
-
Tobacco usage has been linked to increased ROS production and OS. Studies have shown a 48 % increase in seminal leukocyte concentration and a 107 % increase in seminal ROS levels in smokers [39]. Decreased levels of antioxidants (specifically vitamins C and E) have likewise been observed in the seminal plasma of smokers [40, 41]. Smokers are also at a greater risk for DNA fragmentation than nonsmokers [39, 42, 43].
-
Diet and alcohol consumption affect ROS production. It is well established that the accumulation of adipose tissue in obesity leads to the release of pro-inflammatory cytokines, increased testicular temperature, and increased ROS generation by leukocytes [44–47]. Unhealthy diets , such as the antioxidant-deficient diet associated with excessive alcohol consumption, have also been linked to OS. Ethanol consumption has been shown to increase ROS production as well [15, 48–50].
-
Environmental influences such as chemicals, ionizing radiation, and UV light exposure have all been linked to OS. Phthalates (plasticizers found in commonplace items such as food packaging and medical devices), pesticides, and heavy metals lead to cellular and DNA damage via OS [51–56]. Drugs, such as aspirin, increase enzymatic activity which can promote OS. Even in widely used methods, such as assisted reproductive technologies (ART), an increase in the likelihood of OS is observed as spermatozoa are exposed to centrifugation techniques, among others, while seminal antioxidants are removed during washing and cryopreservation [11–13, 57].
-
Natural immune response that results from pathological infection, for example inflammation via leukocytes and macrophages, promotes OS [58, 59]. This can be observed in genital tract infections or current and past Chlamydial infections [60–62]. Prolonged periods of ischemia followed by spontaneous or surgical restoration of blood flow can furthermore lead to increased activation of leukocytes in the testicles thereby resulting in OS, germinal cell necrosis, and ultimately subfertility or infertility [15, 63, 64].
-
Exercise is a source of stress that requires the body to produce large amounts of energy. With the resulting increase in metabolism, the increase in ROS production and decrease in antioxidant levels is inevitable [27–30]. This theory will subsequently be explored in further detail during the latter parts of this chapter.
The Effects of ROS and OS on Fertility
Mechanism of Cell Injury Due to OS
Cellular dysfunction can be caused by OS through any or a combination of the following mechanisms (as shown in Fig. 18.3): lipid peroxidation, DNA damage, redox-dependent signaling pathways, and apoptosis.

Fig. 18.3
Possible mechanisms of ROS/OS induced decrease in sperm function, DNA damage, and fertility. ROS reactive oxygen species, RNS reactive nitrogen species, LPO lipid peroxidation, G6PD glucose-6-phosphate dehydrogenase, NADPH nicotinamide adenine dinucleotide phosphate
As mentioned previously, PUFA present in cell membranes are especially susceptible to oxidative insult resulting in lipid peroxidation. Break down or peroxidation of these fatty acids leads to loss of membrane fluidity as well as the formation of various oxidatively modified products which are toxic to cells [7, 65]. Phosphodiester backbones and DNA bases are some of the other sites that are primarily susceptible to OS. In this instance, ROS-mediated peroxidative damage results in DNA fragmentation and cellular damage.
Increases in ROS have been observed to induce redox-dependent signaling, thereby modifying the activity of various intracellular signaling molecules and pathways. Such pathways can activate tyrosine kinases, which inhibit tyrosine phosphatase activity, while modifying the activity of the mitogen-activated protein kinases (MAPK). Some of these kinases such as p38 MAPK, c-Jun N-terminal kinase (JNK), and extracellular-signal-regulated kinases (ERK) [66] may initiate a chain of reactions that ultimately lead to apoptosis [67]. Studies have also linked the acceleration of the process of apoptosis to ROS-induced DNA damage [68].
Effects of OS on Female Fertility
Female infertility is most commonly observed to be of somatic cell origin and has an endocrine involvement , often amenable to pharmacological treatment [69, 70].
Various reviews have associated reproductive oxidative toxicity to electron transfer–ROS–OS associations [71]. The excess of free radicals in the female reproductive system can lead to reproductive pathologies of the genital tract [72]. Studies have found definite links midst the onset of OS and the development of endometriosis , tubal factor infertility, polycystic ovarian disease, unexplained infertility, adverse embryonic development, preterm labor, and recurrent pregnancy loss (see Fig. 18.4) [72–76]. OS in the female reproductive tract can also severely affect oogenesis and embryo development. These aforementioned pathologies are attributed to various mechanisms including lipid peroxidation, DNA damage, inhibition of protein synthesis, mitochondrial alterations, and depletion of ATP [77–79].

Fig. 18.4
The effect of oxidative stress on fertility
Effects of OS on Male Fertility
The presence of free radicals in semen samples was reported as early as 1943 when a loss of sperm motility was observed in an oxygenated medium [80, 81]. This effect could be alleviated by the addition of catalase , an antioxidant enzyme, responsible for the reduction of hydrogen peroxide. OS was thus identified as the causative factor for the adverse effects displayed by spermatozoa [80].
The most commonly observed cause of male infertility is defective sperm function. With regard to idiopathic infertility , most men who are categorized as infertile present with sufficient amounts of spermatozoa and have normal levels of endocrine function, but the gametes that are produced are often morphologically flawed [80]. Spermatozoa and gametes seem to suffer multiple defects that researchers attribute to the influence of OS (shown in Fig. 18.4) [82]. Inept characteristics of spermatozoa include impaired motility, impaired ability to penetrate the cervical mucus as well as the inability to timeously acrosome react, bind to the zona pellucida, and fuse with the oocyte’s membrane [83–85]. The lipid peroxidation and axonemal protein phosphorylation associated with OS have been suggested as possible mechanisms for decreased sperm motility [86].
Another major contributing factor is the lack of naturally occurring antioxidant enzymes in the cytoplasm of spermatozoa [87]. Mitochondria are located in the spermatozoa midpiece and are generally responsible for oxidative phosphorylation that energizes the spermatozoon and generates ROS as a by-product. Mitochondrial DNA, when compared to nuclear DNA, is 10–17 times faster in accumulating mutations and polymorphisms. Such mutations may also result in ultra-structural defects and abnormal sperm morphology [88–91]. Free radicals are able to attack DNA at multiple points, that is, the pyrimidine and purine bases as well as the deoxyribose backbone. If DNA damage accumulates, it eventually leads to poor blastocyst formation in vitro and higher rates of miscarriage [9, 92–94].
Exercise and OS
The benefits of exercise as a preventative method and/or treatment for metabolic syndrome are hard to ignore. Though exercise contributes to physical and psychological health, intensive strenuous exercise has been linked to infertility in many studies as discussed below. It appears that once a certain threshold of exercise volume is reached, clinical changes in both hormonal levels and fertility parameters are observed [95, 96]. The stress from excessive exercise has been shown to increase ROS levels in the seminal plasma of the male reproductive system . ROS is created as a by-product of aerobic exercise and respiration and oxygen consumption increases 20-fold from rest to high activity. It is thus postulated that ROS can leak from the mitochondria due to elevated oxygen flow through the mitochondrial electron-transport pathway as muscle-based aerobic metabolism produces large amounts of ROS [97]. Raised temperatures, heat stress, and dehydration may lead to amplified rates of oxidative DNA damage in germ cells and hence more mutations in the resulting spermatozoa [98]. Exercise has also been correlated with leukocyte activation and the promotion of OS [24]. The observed link of excessive exercise and infertility may thus be directly attributable to OS. A related mechanism of action is believed to adversely affect the female reproductive system . There is, however, some debate as to exactly how and to what extent this takes place and as to how the difference in the hormonal profiles of the different genders affects the oxidative profile of the body [99].
Studies conducted on males have demonstrated that high levels of endurance cycling (40 min/day at least 3 days/week) negatively affect sperm morphology [100]. A cross-sectional study evaluated the difference between the hormonal profiles of endurance-trained athletes (runners) and resistance-trained athletes (weight lifting) and found that plasma levels of total testosterone and serum levels of free testosterone were significantly lower in both these types of exercise as compared to the controls. Sperm density, motility, and morphology were significantly lowered in the endurance group, while sperm penetration of the cervical mucus was significantly lowered in the resistance group [101]. When high-mileage runners (108.0 ± 4.5 km/week) were compared to moderate mileage (54.2 ± 3.7 km/week) and sedentary control groups, the high-mileage group demonstrated decreased levels of total testosterone and free testosterone in addition to altered sperm count, density, motility, increased populations of immature and round cells, and decreased penetration of bovine cervical mucus. These adverse effects were not seen in the moderate-mileage group, and the researchers suggested that a “volume-threshold” effect of training could be present with high volumes of endurance running [102]. A study compared long-term moderate-intensity treadmill running (∼ 60 % maximal oxygen uptake- VO2max) and high-intensity treadmill running (∼ 80 % VO2max) over a 60-week period integrating five sessions per week of exercise, each session lasting 120 min. The study evaluated the hypothalamus–pituitary–testis (HPT) axis by monitoring levels of sex-hormone-binding globulin (SHBG), luteinizing hormone (LH), and follicle-stimulating hormone (FSH) responses to gonadotropin-releasing hormone (GnRH). Long-term high-intensity training was associated with declined sperm parameters after 24 weeks of exercise and both high- and low-intensity groups showed decreased serum- and free testosterone levels and decreased levels of LH and FSH after 12 weeks of exercise. SHBG increased in both groups after 12 weeks of exercise, while blunted LH and FSH responses to GnRH were observed in both groups. These disturbances were rectified after a 36-week recovery period. The group concluded that long-term strenuous treadmill exercises have a deleterious effect on reproduction. They proposed a mechanism of increased cortisol secretion during training mobilizing fuel stores and subsequently inhibiting GnRH secretion in addition to directly inhibiting the HPT axis function at the hypothalamic–pituitary level as well as inhibiting Leydig cell function via corticotropin-releasing hormone (CRH) receptors, the effect being an altered HPT axis and manifesting as a decrease in LH, FSH, and testosterone levels [103]. Therefore, when examining exercise in relation to male fertility, the intensity, type, and amount of exercise are important.
Acute exhaustive training in humans (pedaling > 60 rev/min, VO2max until exhaustion) was linked to a 26 % increase in plasma lipid peroxides as markers of OS, while moderate- to low-intensity training (< 70 % VO2max) decreased lipid peroxides by 10.3 % [25]. In an attempt to explain this phenomenon, some studies have suggested that mitochondria may play less of a role than initially thought and that heme proteins might play more of a determining role in the onset of OS [26]. Other factors, such as the decreased testosterone and antioxidant (e.g., glutathione) levels may result in increased levels of immature spermatozoa which may stimulate ROS production due to incomplete cytoplasm extrusion [104, 105].
In vivo animal studies investigating the link between infertility , exercise, and OS reported some interesting results. Acute exhaustive running (running 17 m/min until exhaustion) was tested in rats and correlated with increased erythrocyte lipid peroxidation and leukocyte activity within a 24 h-follow-up period [24]. A study using Wistar rats investigated the link between intensive chronic exercise and OS in the male reproductive system . When the experimental group, subjected to intensive swimming exercises, was compared to the control group, a decrease in both plasma testosterone and LH was observed. Furthermore, a detrimental effect on spermatogenesis was noted, elevated levels of lipid peroxidation were found, and the antioxidant activities of superoxide dismutase, catalase, and glutathione were decreased [106]. The authors believed these observations to be responsible for decreased reproductive activities such as steroidogenesis and spermatogenesis . Similar results were found in a study done by the same group examining the effects of different gradients of exercise on subjects. The study reported that the most pronounced results were found in the group subjected to the highest level of exercise [107]. Another study compared sedentary mice to mice exposed to a lifelong regular running plan (1.75 km/day). The study found that running protected against age-related histological changes as well as age-related OS. Running was also associated with fewer biomarkers for lipid peroxidation and protein oxidation, while sedentary mice had elevated levels of antioxidants. The study suggested that this was a mechanism to counteract the oxidative damage that comes with age. The results from these studies therefore clearly suggest that while intensive/exhaustive exercise is detrimental to reproductive health properly sustained amounts of exercise can help reduce the risk of OS [108].
Comparable results were found in human studies. When comparing OS biomarkers and antioxidant levels in elite athletes to recreationally active men, recreationally active men presented with elevated levels of antioxidants. These results pointed to them developing better antioxidant defenses compared to elite athletes. This observation was also accompanied by reduced biomarkers of OS and decreased rates of sperm DNA fragmentation [109]. Another study compared elite athletes (regular 2 h sessions of high-intensity endurance training for 4–5 days per week, 180–190 beats/min, and a maximum oxygen consumption of 58–67 mL/min/kg), recreationally active men (low-to-moderate intensity recreational physical activities, 127–132 beats/min, and a maximum oxygen consumption of 47–53 mL/min/kg), and sedentary men (maximum oxygen consumption of less than 37 mL/min/kg). The study found elite athletes to have a higher number of seminal OS biomarkers (seminal 8-Isoprostane, ROS, and malondialdehyde (MDA), and lower levels of antioxidants (super oxide dismutase, catalase, and total antioxidant capacity) than both of the other groups. The recreationally active men had higher levels of antioxidants and lower amounts of OS biomarkers compared to both elite athletes and sedentary, non-active males [110].
The effects of OS, as well as the adverse effects of excess exercise , on the female reproductive system have been well documented. Excess exercise has been associated with delayed menarche, primary and secondary amenorrhea, oligomenorrhea, and decreased fertility even prevalent when using in vitro fertilization as treatment. Such occurrences are especially prevalent among athletes , vary with athletic discipline and level of competition, and are primarily attributed to hypothalamic dysfunction and disturbances in GnRH pulsatility but also to increased levels of stress and energy imbalances [22, 111–114].Studies conducted on the oxidative profile of females that exercise, however, seem to be in much lesser quantity and far less conclusive about which OS associated mechanism is responsible for these adverse effects [99]. This observation could be explained by the reluctance of researchers to work with female subjects due to the increased variability (as observed during the menstrual cycle) associated with the hormonal differences between men and women. Studies have found that females have lesser levels of OS and higher levels of antioxidant enzyme activity at rest compared to men [115–117]. Studies have also associated acute exercise with differences in vitamin C, E, and glutathione levels amid males and females [118]. Estrogen has also been linked to several antioxidant properties in both in vitro and in vivo studies and some researchers have postulated that this proves females to be less prone to OS and thus explains their longer life expectancy [119–122]. In response to these claims, many research groups have investigated the significance in exercise-associated OS between men and women and found no such difference [113, 114, 118, 123, 124]. Though the evidence of female reproductive irregularities due to exercise induced OS seems to be less pronounced and rather inconclusive, researchers still maintain that the most probable mechanism of action is induced via OS [99].
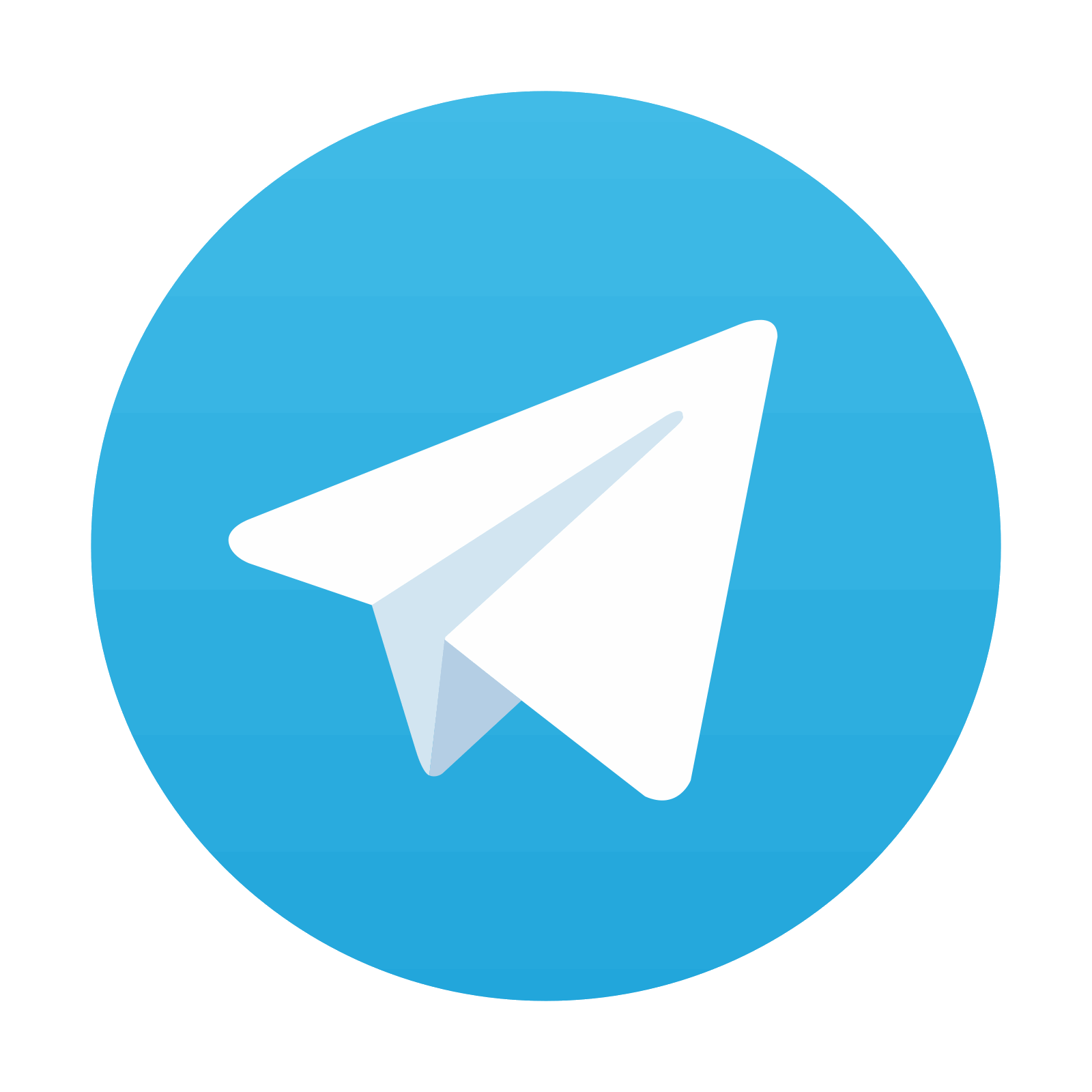
Stay updated, free articles. Join our Telegram channel
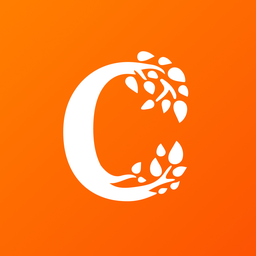
Full access? Get Clinical Tree
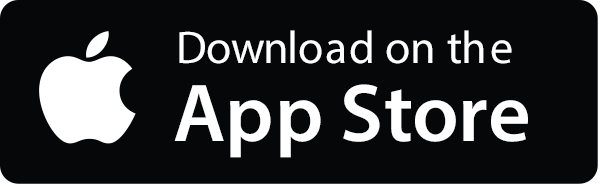
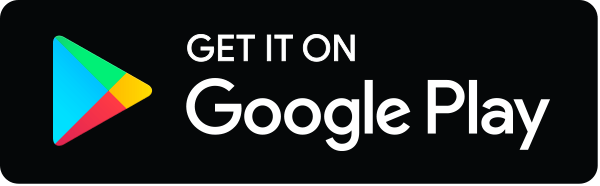