FIGURE 5.1 Glucose homeostasis. Abbreviation: FAO, fatty acid oxidation.
Impact on Cerebral Metabolism
The brain exhibits age-specific differences in its response to hypoglycemia. While the mature brain is quite vulnerable to the effects of hypoglycemia, the immature brain appears to resist the deleterious effects of low BG concentrations under normal conditions. Physiologic mechanisms that facilitate this in the immature brain include the ability to use alternate fuels such as lactate and ketones as well as increases in CBF and extraction of glucose from blood into brain (7). However, even in the immature brain, symptomatic, prolonged, or recurrent severe hypoglycemia can result in neurologic injury, especially in the setting of hypoxia and ischemia. Insulin-induced hypoglycemia might be more dangerous to the immature neonatal and pediatric brain than spontaneous hypoglycemia due to the lack of available alternative fuels such as ketones and fatty acids in the former state. The resulting energy failure mediates neurona damage via glutamate induced excitotoxicity, accumulation of oxygen free radicals, and activation of cellular apoptosis. Long-term sequelae of severe prolonged hypoglycemia in the neonatal period include mental retardation, seizures, or both. Recurrent episodes of severe hypoglycemia may be associated with mild neurocognitive dysfunction, memory deficits, and subtle changes in personality. Pathologic changes corresponding to severe prolonged hypoglycemia include cerebral atrophy, decrease in myelination of cerebral white matter, and gyral atrophy, with parietal and occipital lobes being the most severely affected (8). It is unclear at this time how hypoglycemia affects the brain in older children during critical illness. Preliminary studies suggest that hypoglycemia in older children with critical illness may be associated with higher mortality, worsening of organ failure, and greater ICU lengths of stay. The short-term and long-term consequences of critical illness hypoglycemia (spontaneous or insulin-induced) on the pediatric brain are unknown (9).
Hyperglycemia During Critical Illness
In the setting of critical illness, hyperglycemia develops principally through a combination of: (1) an increase in gluconeogenesis (relative to glucose clearance), and (2) insulin resistance affecting cellular uptake of glucose. Figure 5.2 depicts the pathogenesis of hyperglycemia during critical illness. The principal mechanisms appear to involve counterregulatory hormones (epinephrine, norepinephrine, glucagon, cortisol, growth hormone) and proinflammatory cytokines (tumor necrosis factor alpha, interleukin 1, interleukin 6). Additionally, proinflammatory cytokines may directly inhibit insulin secretion. The overall effect of hyperglycemia in critical illness is to increase BG concentrations and provide a ready source of fuel for vital organs in the body at a time of increased metabolic demand (10). Although hyperglycemia may represent an adaptive response by the body during the acute phase of illness to improve the likelihood of survival, persistence of hyperglycemia during chronic illness may be harmful. Under normal conditions, elevated BG concentrations stimulate the secretion of insulin by the pancreas, which in turn blocks hepatic glucose production and stimulates glucose uptake by the liver, muscle, and adipose tissue. The GLUT family of proteins regulate peripheral glucose uptake via facilitated diffusion. Typically, acute elevations in BG concentrations downregulate insulin-independent GLUT-1, GLUT-2, and GLUT-3 to prevent cellular glucose overload (11). Critical illness causes an overexpression of these transporters, leading to glucose overload and toxicity in organ systems that express these transporters. The upregulation of these insulin-independent GLUTs occurs in the central and peripheral nervous systems as well as in renal tubules, gastrointestinal mucosa, and endothelial, hepatic, and immune cells.
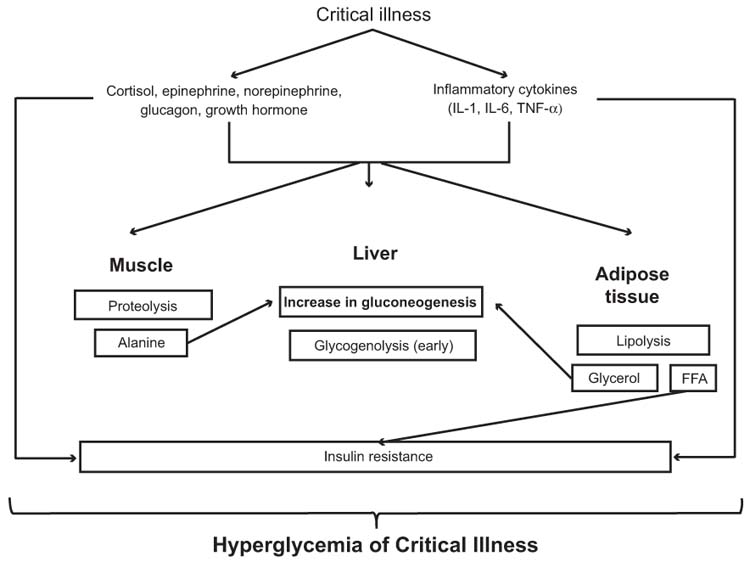
FIGURE 5.2 Pathogenesis of hyperglycemia of critical illness. Abbreviations: FFA, free fatty acids; IL-1, interleukin 1; IL-6, interleukin 6; TNF-α, tumor necrosis factor alpha.
Impact on Cerebral Metabolism
Glucose overload results in excessive glycolysis and oxidative phosphorylation with increased production of reactive oxygen species such as peroxynitrite and superoxide in the brain. These highly reactive species cause mitochondrial dysfunction and direct neuronal damage via lipid peroxidation, protein carbonylation, and DNA damage (12). Neutralization of nitric oxide by superoxide may also impair vasodilation at the endothelial level, thus compromising microcirculatory perfusion. These alterations in energy metabolism lead to increased apoptosis and, consequently, neuronal failure in critically ill patients. Insufficient cellular autophagy exacerbated by hyperglycemia may worsen cellular damage and delay recovery from critical illness. Hyperglycemia may exacerbate preexisting acidosis and increase availability of glutamate to the ischemic brain. This effect may promote N-methyl-D-aspartate (NMDA) mediated excitotoxicity and lead to additional mitochondrial damage from calcium overload (13). Hyperglycemia in critical illness also impairs macrophage and neutrophil activity and alters complement fixation. Hyperglycemia can worsen the inflammatory state by increasing binding of nuclear factor kappa B, leading to increases in transcription of proinflammatory cytokines. Hyperglycemia may contribute to other abnormalities commonly seen during critical illness, such as endothelial dysfunction, alterations in vascular smooth muscle tone, and abnormalities in coagulation that further worsen the cerebral milieu (11).
Alterations in Protein and Lipid Metabolism
Critical illness predisposes to a state of hypercatabolism with increases in protein turnover and losses, principally from skeletal muscle. Albumin levels often decline during the state of hypercatabolism. In the acute phases of critical illness, there is a net transfer of alanine from muscle to liver to support gluconeogenesis. In critical illness, especially sepsis, the skeletal muscle, lung, and kidney release glutamine for uptake by the liver. The liver utilizes glutamine for gluconeogenesis, ureagenesis, and synthesis of proteins and glutathione. Glutamine stores decline to a greater extent than other amino acids in critical illness. Arginine is another amino acid that decreases during critical illness, especially with burn injury. As the acute phase gives way to the chronic phase, persistence of these changes result in negative nitrogen balance and predisposes to the wasting syndrome of critical illness (14).
Lipid metabolism exhibits numerous alterations during critical illness. Inflammation results in increases in free fatty acids and triglycerides, while levels of high-density lipoprotein cholesterol decline. The increases in catecholamines and cytokines inhibit lipoprotein lipase and reduce extracellular lipolysis by endothelial cells. Simultaneously, upregulation of hormone sensitive lipase enhances lipolysis in adipose tissue to an extent that far exceeds demands. Elevated levels of free fatty acids mediate insulin resistance by affecting phosphorylation. This prevents translocation of GLUT-4 to the cell surface and in turn affects uptake of glucose. During critical illness, the liver is unable to secrete enough very low density lipoprotein to match production of triglycerides. The resulting hypertriglyceridemia can cause lipotoxicity, affect endothelial cell function, and exacerbate the state of inflammation by activating toll-like receptor 4. Increases in inflammatory cytokines coupled with limited intake of cholesterol reduce levels of high-density lipoprotein cholesterol during critical illness which predispose to adrenal insufficiency (15).
Impact on Cerebral Metabolism
Critical illness can impair neuronal energetics and predispose to glutamate excitotoxicity even when glutamate is present in physiologic concentrations. Additionally, high levels of nitric oxide generated during the glutamate-glutamine cycle can also cause toxicity and energy failure (16). Hypertriglyceridemia increases oxidative stress and impairs NMDA mediated hippocampal long-term synaptic potentiation. Hypertriglyceridemia affects the ability of leptin to cross the BBB. Since leptin enhances cognition, this could result in cognitive impairments. Additionally, elevated triglyceride levels also modify the release of feeding peptides, many of which affect cognition, via nitric oxide dependent pathways. Collectively, these changes in lipid metabolism predispose to memory loss and cognitive deficits (17).
■ NUTRITION SUPPORT AND GLUCOSE MANAGEMENT
Nutrition support with particular attention to glucose management forms an important aspect of pediatric neurocritical care. This section will cover common conditions in pediatric neurocritical care such as traumatic brain injury (TBI), stroke, hypoxic-ischemic encephalopathy, neuromuscular disorders, and spinal cord injury (SCI). Early provision of enteral nutrition with adequate calories and protein is associated with improved outcomes in adults and children with neurocritical illness. Glucose management to avoid hyperglycemia and hypoglycemia is also very important to improve clinical outcomes. It is less clear at this time if tight glucose control with intensive insulin therapy results in improved outcomes in pediatric neurocritical care.
General Principles
The hypermetabolic stress response in these children places enormous and often variable demands on energy. The body responds to this state with glucose intolerance, dyslipidemia, and protein losses, resulting in negative nitrogen balance and wasting with loss of lean body mass. Supply of protein and glucose during the hypermetabolic state does not necessarily reduce protein breakdown and nitrogen loss. However, net protein balance may improve with protein synthesis. Various nutritional deficiencies can develop during this phase and result in further worsening of critical illness. An anabolic phase follows the hypermetabolic state of critical illness in children who recover. Nutrition support is essential during this recovery phase to improve outcomes.
Assessment of Nutritional Status
Estimates suggest that 1 in 4 children is malnourished at the time of admission to the ICU. Nutritional status of the child with neurocritical illness requires careful assessment at admission and at regular intervals during the course of the ICU duration. Anthropometry is often challenging to perform and difficult to interpret in these critically ill children. Measures of body composition may be more useful, but are difficult to obtain in this population with the possible exception of bioelectrical impedance assessment. Biochemical assessment of visceral protein (albumin, prealbumin, and retinol binding protein) and acute-phase protein (C-reactive protein) pools, nitrogen loss, and energy expenditure are perhaps the most practical to follow in the ICU, but are subject to confounding by a number of variables. Failure to accurately estimate energy needs in children with neurocritical illness can result in both underfeeding and overfeeding, with undesirable consequences. Methods such as indirect calorimetry perform better than predictive clinical energy equations to measure energy expenditure and prescribe appropriate nutrition support in this population (18).
Nutrition Support
The energy burden imposed by the stress of critical illness is often difficult to estimate accurately and varies depending on the phase of illness. Nutrition support, therefore, requires an individualized prescription with frequent monitoring to avoid both overfeeding and underfeeding. Overfeeding critically ill children is associated with lipogenesis, hepatic steatosis, and liver dysfunction. Additionally, increase in carbon dioxide production results in difficulties with weaning from ventilator support (19). While hypocaloric diets in critically ill adults may be beneficial via protein sparing, the evidence of benefit of permissively underfeeding critically ill children is unclear, especially in the face of preexisting malnutrition.
The provision of dietary protein sufficient to optimize protein synthesis and preserve skeletal muscle protein mass is the most important nutrition intervention in critically ill children. A supply of adequate proteins and energy intake improves protein balance by increasing protein synthesis without affecting protein breakdown due to catabolism. The amount of protein required to maintain a positive nitrogen balance may vary according to the severity of illness. The ideal amount and proportion of amino acids required during critical illness are unknown (18). Carbohydrates and fat should ideally make up nonprotein calories in a ratio of 1.2 to 1.8. Essential fatty acid deficiency may develop during critical illness in the absence of adequate lipid supplementation in the diet (20). Lipids typically constitute about 30% to 40% of the total calories. The practitioner should commence administering lipids at 1 g/kg/day, and gradually increase the dose to 2 to 4 g/kg/day, depending on the observed triglyceride levels. Micronutrients including vitamins and trace elements may also play important roles in critical illness and subsequent recovery. Table 5.1 summarizes protein and energy requirements in critically ill children (18).
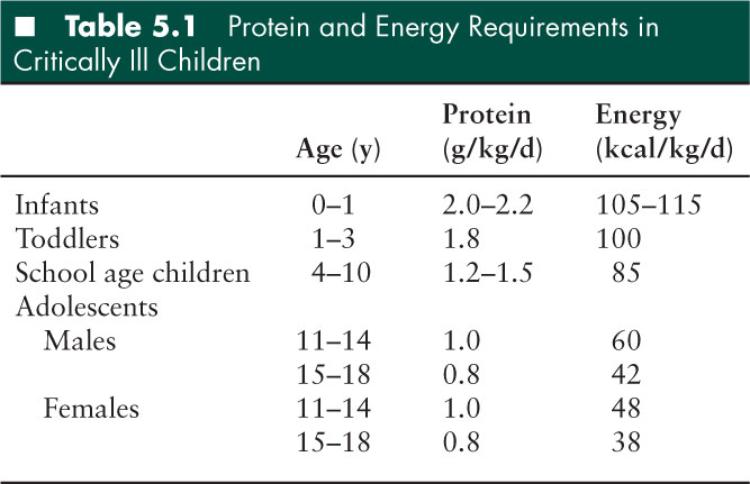
Traumatic Brain Injury
Following TBI in children, mean energy expenditure increases significantly (up to 130%–173% from predicted energy expenditure) and variably between individuals (21,22). Seizures and elevated temperatures are common derangements that occur following TBI and may result in the increased mean energy expenditure observed. Additionally, muscle tone may account for a significant component of this increase in energy expenditure (23). Control of these factors with antiepileptic medications, antipyretic therapies, and sedation with neuromuscular blockade can significantly reduce mean energy expenditure. Most victims of TBI lose weight and attain nitrogen balance only after 4 to 14 days, suggesting the importance of careful nutrition support in enhancing an earlier recovery.
The benefits of commencing early nutrition (whether enteral or parenteral) to reach full nutritional replacement by 7 days following injury appear to be associated with better outcomes in adults with TBI (24). A similar approach may be beneficial in children with TBI, but data are lacking. In those children with TBI who have a functioning gastrointestinal tract, enteral nutrition is preferable to parenteral nutrition for ease of administration, lower cost, and safer profile. However, postpyloric tube feedings and continuous feedings may be necessary to reach energy goals and optimize nutritional status. The use of immune-enhancing diets containing glutamine, arginine, omega-3 fatty acids, and antioxidants is not associated with better outcomes from pediatric TBI (25).
Stroke
Limited data from adult stroke survivors suggest that there is a slight increase in energy expenditure compared to predicted values, but this increase is not sustained (26). Decrease in physical activity and reduction in muscle tone may contribute to these findings. Extensive stroke may be associated with elevated intracranial pressure (ICP) with resulting gastric atony and feeding intolerance. In such circumstances, nasojejunal tube feeding may be necessary to reach caloric goals. Subsequently, after ICP normalizes, it may be possible to switch to nasogastric tube feedings and eventually an oral diet. Dysphagia following stroke may be a major impediment to successful nutrition. In such circumstances, nasogastric or nasojejunal tube feedings are important to optimize nutrition and deliver goal calories. If dysphagia persists, a percutaneous or surgical gastrostomy or jejunostomy tube may be required for continued nutrition support. The risk of reflux of gastrointestinal contents with aspiration remains high if the swallowing mechanism is impaired following stroke. Weaning from tube feedings requires close involvement of speech-language therapists with calorie counts to determine the exact amount of nutrition intake (27).
Hypoxic-ischemic Encephalopathy
Patients with hypoxic-ischemic encephalopathy may have lower energy expenditure. However, intercurrent seizures and autonomic dysreflexia can result in episodic hypermetabolism profound enough to cause severe weight loss and protein breakdown (28). Indirect calorimetry may be invaluable in such circumstances to accurately study energy requirements and prescribe appropriate nutrition therapy. Due to significant neurologic impairments, tube feedings are often required to deliver target calories. Constipation and gastroesophageal reflux are major impediments to successful nutrition in this population. Additionally, overfeeding is a common problem in this group of children.
Neuromuscular Disorders
Children with spinal muscular atrophy often have impaired swallowing mechanisms with limited mouth opening and difficulties chewing due to bulbar dysfunction (29). Critical illness and death is often due to aspiration pneumonia and respiratory failure. While growth failure is common in those with more severe disease, overfeeding and excessive weight gain is common in those with milder forms of the disease. Gastroesophageal reflux, delays in gastric emptying, and constipation commonly complicate the picture further in this group. While nasogastric or nasojejunal tube feedings may overcome some of the problems, percutaneous or surgical gastrostomy or jejunostomy tubes may be superior to consistently deliver nutrition without compromising the delicate respiratory status in these children. During acute illness, these children are more prone to hypoglycemia due to severe muscle wasting (30). Additionally, disorders of fatty acid oxidation may coexist in this group of children rendering them more vulnerable to fasting states (31). Prompt provision of calories and avoidance of prolonged fasting states can prevent muscle breakdown in these children during critical illness.
Guillain-Barré syndrome (GBS) is another entity in the ICU that poses tremendous challenges to nutrition support. Although the patient with GBS is immobile and appears to have reduced energy expenditure, hypermetabolism is common in GBS (32). In addition, patients are often malnourished at admission as bulbar weakness precludes adequate oral intake (33). If nutritional demands are not promptly met, patients rapidly become undernourished and have an increased incidence of pressure sores and infections. Patients with GBS should receive a high calorie (40–45 nonprotein calories/day) and high-protein (2–2.5 g/kg) enteral formula for optimal nutrition. Continuous enteral nutrition is preferable to intermittent or bolus feeding in these patients who also frequently experience delayed gastric emptying with gastroesophageal reflux, constipation, and erosive gastritis.
Spinal Cord Injury
Data from adults experiencing SCI suggest that energy expenditure is reduced acutely (34). Although these patients exhibit a negative nitrogen balance, this negative balance is obligatory, and attempts to correct it by increasing caloric intake may result in overfeeding with its attendant consequences. Indirect calorimetry may provide a more accurate reflection of the patient’s caloric requirements, and could prevent overfeeding by allowing adjustments of intake based on measured energy expenditure rather than predicted energy expenditure. Enteral feeding is preferable to the parenteral route, as it produces a lower incidence of infectious complications and hyperglycemia. Nasogastric or nasojejunal tube feedings may be necessary if swallowing mechanisms are impaired. Percutaneous or surgical feeding tubes are preferable for long-term nutritional support in these patients.
Glucose Management
Hyperglycemia in pediatric critical illness is very common, with an estimated 49% to 72% of children experiencing BG concentrations greater than 150 mg/dL (35–38). Notably, 20% to 35% of critically ill children experience BG concentrations greater than 200 mg/dL. BG concentrations in critically ill children can often peak as high as 283 ± 115 mg/dL. Sustained hyperglycemia is also common over a prolonged period of ICU admission and may persist for up to half the duration of ICU stay. In the last decade, several studies have demonstrated the association of hyperglycemia in critically ill children with mortality (35–38). Specifically, peak BG concentrations and sustained elevation of BG concentrations appear to be associated with mortality. This association of hyperglycemia with mortality consistently appears across different pediatric conditions, including septic shock, burns, TBI, postcardiac surgery, and trauma. Hyperglycemia is also associated with longer periods of ICU and hospital stay as well as more frequent organ failure and nosocomial infections in critically ill children (35–38).
Studies of tight glucose control in critically ill adults have had mixed results with some observing worse outcomes from tight glucose control (39–41). Notably, all studies of tight glucose control in critically ill adults observed significant increases in hypoglycemia. Consequently, the initial rush to embrace this therapy has justifiably given way to a more cautious approach in the critical care community. It is worrying that studies suggest that hypoglycemia is also associated with mortality and worsening organ failure in critically ill children (42). Multicenter studies of tight glucose control in critically ill children are currently underway to answer this important question of whether tight glucose control can improve outcomes in critically ill children experiencing hyperglycemia (43).
Traumatic Brain Injury
Studies in children experiencing TBI have shown that admission hyperglycemia is associated with mortality and poor neurologic outcomes following injury (44,45). Persistent hyperglycemia, especially beyond 48 hours, is also associated with poor neurologic outcomes in children with TBI (46,47). Most studies of tight glucose control in adults with TBI have not observed any benefits, with some observing worse outcomes (48,49). Additionally, there is increasing concern about hypoglycemia and its risks to the injured brain. Studies employing microdialysis in adults with severe TBI have demonstrated increased metabolic stress in the cerebral milieu with tight glucose control compared with less rigorous BG targets (50). The practice of tight glucose control may reduce levels of lactate and ketones, which are likely to be the preferred fuels in the injured brain. The optimal BG target concentration following pediatric TBI remains unknown. It is, therefore, prudent to avoid extremes of hyperglycemia and hypoglycemia in pediatric survivors of TBI.
Stroke
Data from adults experiencing stroke observe that hyperglycemia is associated with worse outcomes (51). However, studies of tight glucose control in adult victims of stroke have not demonstrated any benefits, most likely due to increases in hypoglycemia that might negate any advantages of tight glucose control (52). Extrapolating from adult stroke guidelines, it would be reasonable to target BG concentrations in a more “moderate” range, taking care to avoid extremes of hyperglycemia and hypoglycemia.
Hypoxic-ischemic Encephalopathy
Hyperglycemia is common after cardiac arrest due to the upregulated metabolic state of the post cardiac arrest syndrome and may be associated with poor outcomes (53). However, studies have observed that tight glucose control may not be necessary to improve outcomes, with a more moderate BG target up to 150 mg/dL being clinically comparable (54,55). It is extremely important to avoid hypoglycemia at all costs to prevent further neuronal damage in this state.
Spinal Cord Injury
There is no pediatric data on the association of hyperglycemia with SCI and outcomes. Extrapolating from TBI, it would be reasonable to avoid tight glucose control in this group and target a modest BG range, taking care to avoid hypoglycemia.
■ CONCLUSION
Nutrition support with attention to BG management forms an important aspect of pediatric neurocritical care. Early provision of enteral nutrition with adequate calories and protein is important in children with neurocritical illness. Protocols to ensure adequate nutrition support may improve outcomes in neurocritical care by minimizing energy deficits or excesses. Techniques such as indirect calorimetry, if available, are helpful to provide targeted nutrition support. BG management to avoid hyperglycemia and hypoglycemia is also very important to improve clinical outcomes. Studies are underway to determine if tight glucose control with intensive insulin therapy results in improved outcomes in critically ill children. The results from these studies will help inform practice in pediatric neurocritical care.
■ REFERENCES
1. et al. The [14C] deoxyglucose method for the measurement of local cerebral glucose utilization: theory, procedure, and normal values in the conscious and anesthetized albino rat. J Neurochem.. 1977;28 897–916 .
2. , . Supply and demand in cerebral energy metabolism: the role of nutrient transporters. J Cereb Blood Flow Metab. 2007;27:1766–1791.
3. , . Circulation and energy metabolism et al, eds.Basic Neurochemistry: Molecular, Cellular, and Clinical Aspects. 6th ed. New York, NY: Raven Press; 1999;637–669.
4. et al. Nonoxidative glucose consumption during focal physiologic neural activity. Science. 1988;241:462–464.
5. , . Glucose metabolism in the developing brain. Semin Perinatol. 2000;24:107–115.
6. et al. Cerebral metabolic rate for glucose during the first six months of life: an FDG positron emission tomography study. Arch Dis Child Fetal Neonatal Ed. 1996;74:F153–F157.
7. . Hypoglycemic brain injury. Semin Perinatol. 2001;6:147–155.
8. . Hypoglycemia. In: et al, eds.Nelson Textbook of Pediatrics. 19th ed. Philadelphia, PA: Elsevier Saunders; 2011: 517–531.
9. , , . Hypoglycemia in critically ill children. J Diabetes Sci Technol. 2012;6:48–57.
10. . Stress hyperglycemia in pediatric critical illness: the intensive care unit adds to the stress!. J Diabetes Sci Technol. 2012;6:37–47.
11. . How does blood glucose control with insulin save lives in intensive care? J Clin Invest. 2004;114:1187–1195.
12. , . Glucose neurotoxicity. Nat Rev Neurosci. 2008;9:36–45.
13. et al. Hyperglycemia enhances extracellular glutamate accumulation in rats subjected to forebrain ischemia. Stroke. 2000;31:183–192.
14. . Nutritional therapy in critically ill and injured patients. Surg Clin N Am. 2011;91:579–593.
15. , . Insulin resistance in critical illness. Curr Opin Pediatr. 2011;23:269–274.
16. , . Glutamate in neurologic diseases. J Child Neurol. 1997;12:471–485.
17. et al. Obesity and hypertriglyceridemia produce cognitive impairment. Endocrinology. 2008;149:2628–2636.
18. . Nutrient metabolism and nutrition therapy during critical illness. In: , , eds. Pediatric Critical Care. 4th ed. Philadelphia, PA: Elsevier Saunders; 2011:1073–1088.
19. et al. Parenteral administration of different amounts of branch-chain amino acids in septic patients: clinical and metabolic aspects. Crit Care Med. 1997;25:418–424.
20. , , . Decreased antioxidant status and increased lipid peroxidation in patients with septic shock and secondary organ dysfunction. Crit Care Med. 1995;23:646–651.
21. et al. Nutritional support and measured energy expenditure of the child and adolescent with head injury. J Neurosurg. 1987;67:846–851.
22. , , . Measured energy expenditure in severe head trauma. J Trauma. 1989;29:1633–1636.
23. , , . Determinants of resting energy expenditure in young children. J Pediatr. 1994;125:362–367.
24. et al. Guidelines for the management of severe traumatic brain injury. XII. Nutrition. J Neurotrauma. 2007;24(suppl 1):S77–S82 .
25. et al. Temporal nutritional and inflammatory changes in children with severe head injury fed a regular or an immune-enhancing diet: a randomized, controlled trial. Pediatr Crit Care Med. 2006;7:56–62.
26. et al. Measuring longitudinally the metabolic demands of stroke patients, resting energy expenditure is not elevated. Stroke. 2003;34:502–507.
27. et al. Nutrition in the stroke patient. Nutr Clin Pract. 2011;26:242–252.
28. et al. Severe weight loss and hypermetabolic paroxysmal dysautonomia following hypoxic ischemic brain injury: the role of indirect calorimetry in the intensive care unit. JPEN J Parenter Enteral Nutr. 2008;32:281–284.
29. et al. Consensus statement for standard of care in spinal muscular atrophy. J Child Neurol. 2007;22:1027–1049.
30. et al. Hypoglycaemia in spinal muscular atrophy. Lancet. 1995;346:609–610.
31. et al. Abnormal fatty acid metabolism in childhood spinal muscular atrophy. Ann Neurol. 1999;45:337–343.
32. , , . Hypermetabolism and hypercatabolism in Guillain-Barré syndrome. JPEN J Parenter Enteral Nutr. 1992;16:464–472.
33. , . Guillain-Barré syndrome. In: , ed. Critical Care Clinics: Update on Neurologic Critical Care. Philadelphia, PA: WB Saunders; 1997: 1–15.
34. et al. Acute management of nutritional demands after spinal cord injury. J Neurotrauma. 2011;28:1497–1507.
35. et al. Association of timing, duration, and intensity of hyperglycemia with intensive care unit mortality in critically ill children. Pediatr Crit Care Med. 2004;5:329–336.
36. , . Persistent hyperglycemia in critically ill children. J Pediatr. 2005;146:30–34.
37. et al. Paediatric Study Group Australian and New Zealand Intensive Care Society. Glucose control, organ failure, and mortality in pediatric intensive care. Pediatr Crit Care Med. 2008;9:147–152.
38. , , . Alterations in glucose homeostasis in the pediatric intensive care unit: hyperglycemia and glucose variability are associated with increased mortality and morbidity. Pediatr Crit Care Med. 2008;9:361–366.
39. et al. Intensive insulin therapy in the critically ill patients. N Engl J Med. 2001;345:1359–1367.
40. et al. Intensive insulin therapy in the medical ICU. N Engl J Med. 2006;354:449–461.
41. NICE-SUGAR Study Investigators, , , et al. Intensive versus conventional glucose control in critically ill patients. N Engl J Med. 2009;360:1283–1297.
42. , . Relationship between hypoglycemia and mortality in critically ill children. Pediatr Crit Care Med. 2010;11:690–698.
43. Stress hyperglycemia in pediatric critical illness: the intensive care unit adds to the stress! J Diabetes Sci Technol. 2012;6:37–47.
44. et al. Elevated initial blood glucose levels and poor outcome following severe brain injuries in children. J Trauma. 1991;31:1356–1362.
45. et al. Hyperglycemia and outcomes from pediatric traumatic brain injury. J Trauma. 2003;55:1035–1038.
46. et al. Prognostic factors and outcome of children with severe head injury: an 8-year experience. Childs Nerv Syst. 2002;18:129–136.
47. et al. Relationship between hyperglycemia and outcome in children with severe traumatic brain injury. Pediatr Crit Care Med. 2012;13:85–91.
48. et al. Intensive versus conventional insulin therapy in critically ill neurologic patients. Neurocrit Care. 2010;13:299–306.
49. et al. Intensive insulin therapy in the neurocritical care setting is associated with poor clinical outcomes. Neurocrit Care. 2010;13:307–312.
50. et al. Intensive insulin therapy reduces microdialysis glucose values without altering glucose utilization or improving the lactate/pyruvate ratio after traumatic brain injury. Crit Care Med. 2006;34:850–856.
51. et al. Stress hyperglycemia and prognosis of stroke in nondiabetic and diabetic patients: a systematic overview. Stroke. 2001;32:2426–2432.
52. , , . Insulin for glycaemic control in acute ischaemic stroke. Cochrane Database Syst Rev. 2011;(9):CD005346.
53. , , . Glycemia in the post-resuscitation period. The Cerebral Resuscitation Study Group. Resuscitation. 1989;17(suppl):S181–S188.
54. et al. Strict versus moderate glucose control after resuscitation from ventricular fibrillation. Intensive Care Med. 2007;33:2093–2100.
55. et al. Strict normoglycaemic blood glucose levels in the therapeutic management of patients within 12h after cardiac arrest might not be necessary. Resuscitation. 2008;76:214–220.
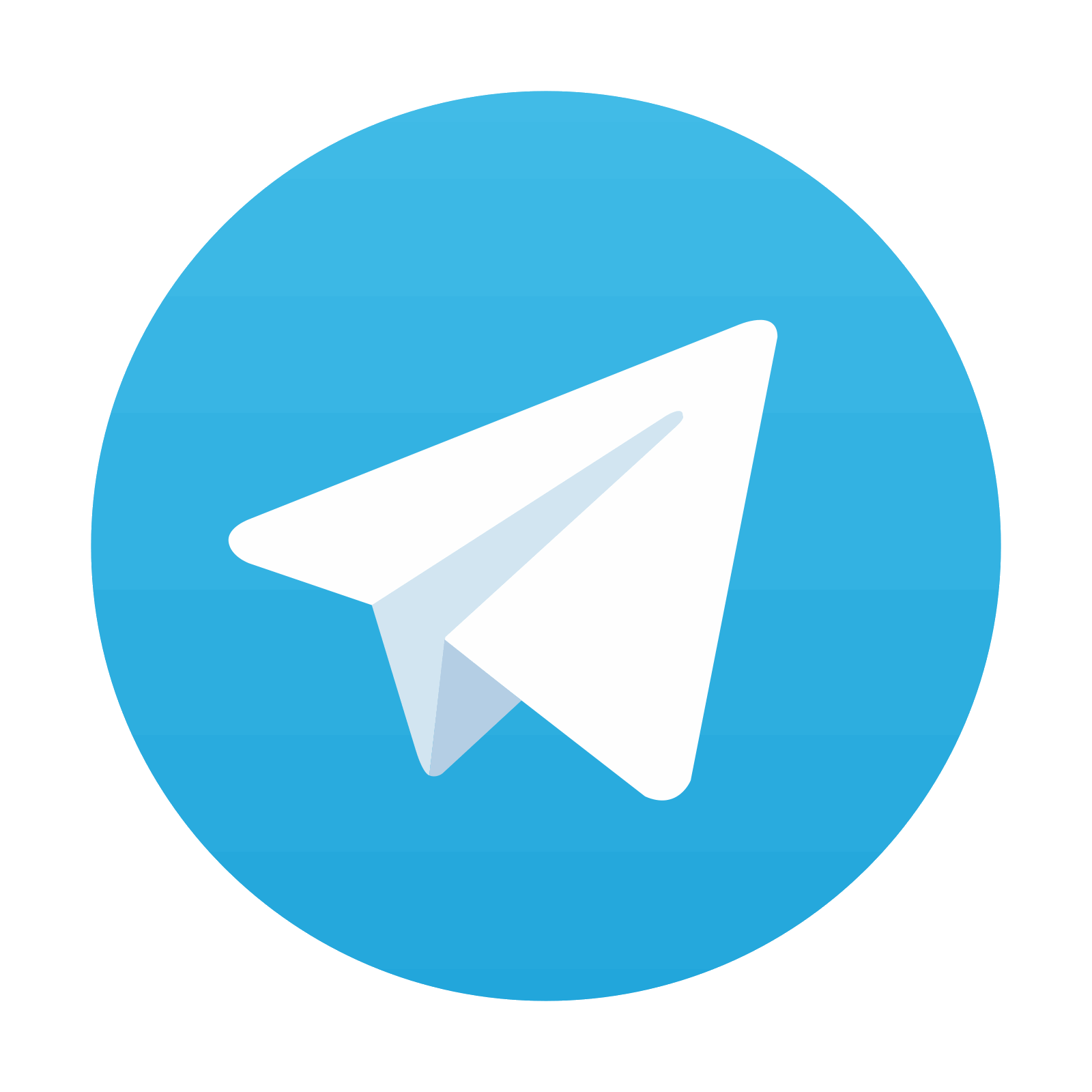
Stay updated, free articles. Join our Telegram channel
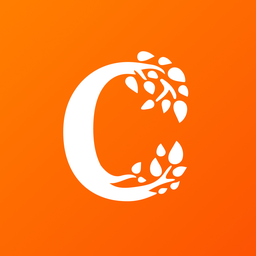
Full access? Get Clinical Tree
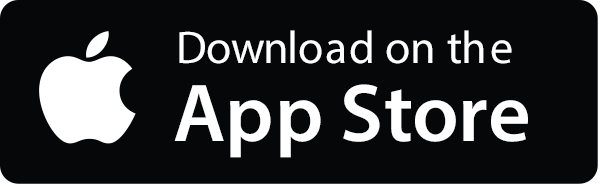
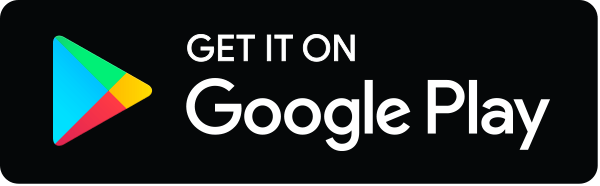