Fig. 7.1
Extracellular matrix (ECM) ligands and receptors. Both ECM ligands and receptors typically possess modular structure, containing domains that allow them to bind to each other as well as a broad range of different molecules. Depicted here are the representatives of (a) proteoglycans (decorin), (b) structural ECM proteins (laminin), and (c) matricellular proteins (CCNs). In addition, different types of ECM receptors, such as (d) integrins, (e) syndecans, and (f) dystroglycans, are included
Table 7.1
Representative extracellular matrix ligands and receptors in the endothelium
Receptor |
Function |
Source | |
---|---|---|---|
Collagens | |||
I |
α 1 β 1 ; α 2 β 1 |
EC morphogenesis; tensile strength of the vessel wall |
|
III |
α 1 β 1 ; α 2 β 1 |
Elasticity of the vessel wall |
|
IV |
α 1 β 1 ; α 2 β 1 |
Structural component of vascular basement membrane |
|
V |
α 1 β 1 ; α 2 β 1 |
Inhibition of EC adhesion and proliferation |
|
VI |
β 1 integrins |
Adhesion of smooth muscle cells |
|
Elastin and microfibrillar proteins | |||
Elastin |
Elasticity of the vessel wall |
||
Fibrillins (1–2) |
α v β 3 ; α 5 β 1 ; α v β 6 |
Structural components of microfibrils |
|
Fibulins (1–7) |
α v β 3 ; α v β 5 ; α 9 β 1 |
Structural components of microfibrils |
|
Laminins | |||
Laminin-411/511 |
α 3 β 1 ; α 6 β 1 ; syndecans; dystroglycan |
Structural component of vascular basement membrane |
|
Fibronectin |
α v β 3 ; α 5 β 1 |
EC migration; vessel lumen formation |
|
Matricellular proteins | |||
Thrombospondins (1–5) |
CD36; IAP; β 3 integrins |
Inhibition of EC adhesion, migration, survival, and tube formation |
|
CCNs (1–2) |
α v β 3 ; α 6 β 1 ; α II bβ 3 ; α M β 3 |
EC adhesion, migration, differentiation, and survival |
|
Tenascins (-C, -R, -X, -W) |
α v β 3 ; syndecan-4 |
EC adhesion, migration |
|
Small leucine-rich proteoglycans | |||
Decorin |
Structural component of vessel wall |
||
Biglycan |
Structural component of vessel wall |
||
Basement membrane proteoglycans | |||
Perlecan |
α 2 β 1 |
Structural component of vascular basement membrane |
Structural ECM Proteins
Collagen constitutes the most abundant component of the ECM, where it provides tensil strength, regulates cell adhesion and migration, and directs tissue development [5]. Its basic building blocks consist of three alpha chains intertwined to form a triple helix, which further assemble into extended fibrilar structures with tissue-specific alignment and distribution. A dramatic diversity of collagen molecules—at least 28 different vertebrate collagens have been reported to date—derive from distinct α-chain composition as well as diverse supramolecular structures [6]. For instance, fibril-forming collagens pack together side-by-side to form thick fibrils, whereas network-forming collagens form open mesh-like structures with various geometries. Vessel walls, especially arterial, can contain up to 17 different collagen types, with collagens I, III, IV, V, and VI having the highest expression levels [7] (Table 7.1).
Another major fibrillar ECM protein is elastin, which imparts the property of elasticity to tissues that undergo repeated stretch, such as the lung, skin, and blood vessels [8]. In fact, elastin is one of the earliest structural matrix proteins to be expressed by vascular smooth muscle cells (SMCs) in large vessels. Similar to collagen, secreted tropoelastin monomers (precursors of elastin) assemble into elastin fibers through cross-linking of their lysine residues and activity of the lysyl oxidase (LOX) enzyme family. In addition, elastin strongly associates with a scaffold of fibrillin-rich microfibrils, which contribute to the integrity of elastin fibers [6].
Laminins are one of the main constituents of basement membranes, where they act as scaffolds necessary for the initial assembly of the membrane. Laminin molecules are heterotrimeric glycoproteins consisting of one α, one β, and one γ chain held together by disulfide bonds [9] (Fig. 7.1b). In vertebrates, different types of each of the chains have been found (α1–5, β1–3, γ1–3), and their expression varies between the cell types and during development; together, they constitute 18 different laminin trimers. The most important laminins found in endothelial basement membranes are LN 411 and LN 511, which contain α4β1γ1 and α5β1γ1 chains, respectively.
Another protein that acts as a scaffold for the assembly of other matrix components is fibronectin, a large dimer molecule that exists in different isoforms and different conformations. In addition to its role in directing the organization of the ECM, fibronectin is essential for cell attachment and migration during both physiological and pathological conditions [10]. Its ability to stretch and expose cryptic sites within its molecule enables fibronectin to elicit pleiotropic effects on cellular behavior and to modulate cellular responses [11].
Matricellular Proteins and Matrikines
Although structural ECM proteins can affect cellular functions through mechanotransduction and through binding to many cell-surface receptors, tissues employ an additional mechanism to further modulate these cell–matrix interactions—matricellular proteins . This fast-growing group of proteins appears not to contribute directly to the organization or physical properties of the ECM, but it rather modulates a broad range of cell regulatory functions through various mechanisms [12]. Typical representatives are thrombospondin (TSP), SPARC (secreted protein, acidic and rich in cysteine), tenascin, osteopontin, and CCN (Cyr61), but numerous other novel proteins such as EGFL7 [13] and MAGP-1 [14] seem to share many of their structural and functional properties. Confirming their nonstructural roles, phenotypes of mice lacking a matricellular protein are either normal or very mild, and typically exacerbated upon mechanical stress and wound healing [15, 16]. Similarly, these proteins are only expressed during embryonic development and in response to wound injury, tissue remodeling, inflammation, cancer, and other chronic diseases [17, 18]. Their structure is typically modular, containing domains that are able to bind a variety of other ECM proteins, cell-surface receptors, growth factors, cytokines, and proteases, enabling them to control an array of cellular functions in a context-dependent manner (Fig. 7.1c). In the endothelium, matricellular proteins exert either pro- or antiangiogenic effects, affecting virtually every phase of blood vessel formation (see the ‘ECM and Blood Vessel Growth’ section).
Finally, both structural and nonstructural ECM proteins undergo partial proteolytic cleavage, generating fragments—known as matrikines—that also exert effects on cellular functions. Typical examples include angiostatin derived from plasminogen, endostatin from collagen XVIII, restin from collagen XV, and anastellin from fibronectin, as well as SPARC- and TSP-derived fragments. These bioactive fragments play an active role in angiogenesis by affecting different steps of the angiogenic process and by employing different mechanisms of action [19].
7.1.2 ECM Receptors
Integrins
Structurally and functionally diverse ECM proteins exert their effects through different cell-surface receptors, the most important of which are integrins. This is a group of large heterodimeric transmembrane glycoproteins that is highly conserved throughout evolution, ranging from sponges to humans. Integrin receptors consist of noncovalently associated α and β subunits, and each subunit contains an extracellular domain, a single transmembrane region, and a short cytoplasmic region [20] (Fig. 7.1d). Together, 18α and 8β subunits can form 24 different integrin heterodimers, each specific for a unique set of ECM ligands.
Rather than just simple adhesion molecules, integrins act as complex relay points that transmit bidirectional signals across the plasma membrane. Upon ligation, these receptors change their conformation from inactive to active, and cluster together to form large signaling hubs, which further mediate the effects of the extracellular environment on cell proliferation, motility, survival, and other processes. In contrast, integrins are also able to respond to signals coming from inside the cell and engage in so-called inside-out signaling; this further allows the cells to control the affinity and avidity of integrin receptors for their ligands (for extensive review of integrin signaling see Hood and Cheresh [21]).
The most important vascular integrins that are expressed on the quiescent endothelium include α1β1, α2β1, α3β1, α6β1, and α6β4, which predominantly bind to collagens and laminins, but also α5β1 and αvβ5, which bind to fibronectin and vitronectin, respectively. In remodeling endothelium, in contrast, α5β1 and αvβ3 undergo a dramatic increase in expression and exert diverse functions through binding an assortment of ECM ligands [22].
Other ECM Receptors
In addition to integrins, ECM proteins can signal through previously mentioned cell-surface PG syndecans. These are single transmembrane proteoglycan molecules that carry heparan sulfate and chondroitin sulfate chains, which facilitate interactions with a diverse group of proteins including different growth factors and ECM proteins such as fibronectin and laminin [23] (Fig. 7.1e). In addition, syndecans act as co-receptors that are able to further modify the activity of integrin receptors.
Finally, dystroglycans have been characterized as novel nonintegrin receptors able to bind laminins and mediate their effects [24]. They consist of a highly glycosylated α chain noncovalently anchored to a transmembrane β chain (Fig. 7.1f). Although their function still remains elusive, dystroglycans are upregulated in endothelial cells (ECs) during both physiological and pathological angiogenesis .
7.2 ECM and Blood Vessel Growth
7.2.1 Introduction
Growth of new blood vessels proceeds through two main mechanisms: vasculogenesis, which entails differentiation of mesodermal cells into angioblasts and their subsequent fusion into a primitive vascular plexus, and angiogenesis, or formation of new blood vessels from the pre-existing blood vessels [25, 26]. The former predominantly occurs during embryonic development, although certain pathological processes such as cancer involve incorporation of circulating progenitors into the vascular network; the latter process defines vessel growth not only in the adult but also during the later stages of vascular plexus remodeling.
Angiogenic sprouting ensues through a well-defined cascade of events, and its underlying molecular machinery has been extensively studied over the last decade. Angiogenic stimuli activate quiescent ECs, which then assume a hierarchical organization into highly motile tip cells and trailing stalk cells. Tip cells extend numerous filopodia, sense the environment, and lead the sprout into a certain direction while stalk cells actively proliferate and concurrently form vascular lumens. When tip cells from opposing sprouts meet, they lose their invasive phenotype and generate tight junctions, allowing formation of continuous, patent vessels and promoting their subsequent maturation. Such complex and tightly regulated events are under control of an intricate network of different molecular players spearheaded largely by the vascular endothelial growth factor (VEGF)/Notch signaling axis, which is extensively reviewed elsewhere [27]. However, angiogenic sprouting does not occur in isolation but within an elaborate fabric of ECM cues that guide cellular interactions with the surrounding environment (Fig. 7.2). Therefore, this chapter will focus on how ECM components regulate different phases of vessel growth.

Fig. 7.2
Extracelluar matrix (ECM) drives physiological angiogenesis. At the onset of angiogenic sprouting, blood-borne proteins such as fibronectin, vitronectin, and fibrinogen extravasate through leaky vasculature into the perivascular space and interact with the pre-existing ECM to form a provisional matrix. In addition, the basement membrane gets degraded, exposing the endothelial cells (ECs) to the provisional matrix and proteins such as collagen I, which is abundantly present in the interstitial space. The activity of the ECs matches the changes in the extracellular environment, resulting in the upregulation of proangiogenic factors such as αvβ3 and α5β1 integrins. Furthermore, ECs secrete various ECM proteins that support different phases of the angiogenic process
7.2.2 Resting Vasculature
ECs that line the walls of blood vessels in an organism are typically quiescent and packed tightly enough to facilitate the integrity of the vessel but permeable enough to enable flux of material between blood and interstitium; different combinations of tight junctions and adherens junctions, through which ECs interact with each other, regulate this permeability in a tissue-specific manner [28, 29]. However, much of the EC surface is in contact with a vascular basal membrane comprising laminins, collagen IV, nidogen, perlecan, and other PGs, which together assemble into a matrix that insulates ECs from the surrounding interstitial space. Another type of vascular cells that further regulate EC function are mural cells—smooth muscle cells in arteries, arterioles, and veins; and pericytes in capillaries and venules [30]. They interact intimately with the surface of ECs, share the same basement membrane, and actively contribute to vessel stabilization and maturation as well as regulation of the blood flow.
Master regulators that keep ECs in the quiescent state include homeobox family members such as Hox A5, Hox D10, Hox C9, and Gax [31–34]. They reinforce a specific gene-expression program, downregulating genes necessary for EC migration and proliferation, and upregulating genes with antiangiogenic activities. Although these mostly include various growth factors and signaling molecules, members of the ECM directly promote EC quiescence, as shown in the ‘EC Stabilization and Maturation(Vessel Stabilization and Maturation)’ section.
7.2.3 Endothelial Cell (EC) Activation
The initial phase of vessel growth encompasses simultaneous changes in the extracellular environment and matrix composition on the one hand, and EC activity on the other. Although a spectrum of different growth factors and bioactive molecules promote angiogenesis, VEGF are the best-studied growth factors able to initiate these early-signaling events leading to new vessel sprouting. They bind to their receptors on the surface of ECs and activate members of Src family kinases, which then phosphorylate vascular endothelial (VE)-cadherin and promote junction disassembly and vessel permeability [35]. In addition, FAK kinase phosphorylates β-catenin and facilitates VE-cadherin–β-catenin dissociation [36]. This allows blood-borne ECM proteins, such as fibronectin, vitronectin, and fibrinogen, to extravasate into the perivascular space, interact with the pre-existing ECM, and form a ‘provisional ECM’, which facilitates all the subsequent phases of vessel growth.
These events are perfectly orchestrated with the changes in EC morphology and behavior. Namely, in addition to affecting EC junctions and EC communication, angiogenic signals coordinate simultaneous deactivation of quiescence-maintaining Hox genes and induction of proangiogenic members of the Hox gene family, such as HoxB3, HoxD3, and HoxA9 [37–39], which promote invasive EC phenotype [39]. For instance, activated ECs rapidly change the repertoire of integrin receptors on their surface, dramatically upregulating αvβ5, α5β1 and, in particular, αvβ3 integrin, which preferentially bind components of the provisional ECM [22]. In addition, Hox genes control the expression of different proteases as well as Eph receptors, which further regulate sprout formation. Taken together, changes in EC phenotype upon angiogenic signaling match the dynamics of remodeling ECM, enabling the cells to sense and respond to the new matrix topology.
7.2.4 Tip/Stalk Cell Selection
As mentioned in the introduction, upon activation ECs establish a hierarchy of leading tip cells and trailing stalk cells. Although it is well established that VEGF-induced Dll4 upregulation in tip cells and activation of the Notch pathway in the stalk cells define tip/stalk cell phenotypes, there has been evidence that ECM plays a role in tip cell selection. Namely, Estrach et al. demonstrated that ligation of α2β1 and α6β1 integrins by laminin-111 induces FoxC2 signaling and high Dll4 expression [40]. Moreover, Lama4 mutant mice display excessive filopodial branching and tip cell formation as a result of decreased Dll4 signaling [41]. Finally, gene expression profiling of ECs enriched for tip cells revealed laminin β1 as one of the upregulated genes, reinforcing the hypothesis that basement membrane components such as laminins could have additional functions in addition to their role in vessel maturation and stabilization [42]. In addition, other extracellular-associated proteins were shown to directly affect Notch signaling. Namely, both EGFL7 and MAGP-2 act as Notch antagonists in ECs, suggesting they may actively participate in regulating the balance between tip and stalk cells within the angiogenic sprout [43–45].
7.2.5 EC Invasion and Migration
To lead the sprout in the right direction, tip cells interact with and modify the surrounding matrix. Therefore, a group of factors specifically enriched in these cells involves ECM degrading enzymes such as cathepsin S, a disintegrin and metalloproteinase with TSP motifs (ADAMTS), and urokinase-plasminogen-activated receptor (uPAR) [42]. These proteases release growth factors which are stably deposited in the ECM through binding other proteins and PGs. For instance, both VEGF and basic fibroblast growth factor (bFGF) associate with heparan-like glycosaminoglycans and require haparinases and proteases to become fully active [46]. In addition, plasminogen activators release transforming growth factor (TGF)-β, which then further promotes expression of angiogenic signals and ECM-degrading proteases [47].
Furthermore, proteases participate in the degradation of the basement membrane and remodeling of the surrounding matrix, thus regulating EC migration on multiple levels [48]. Namely, disassembly of the basement membrane exposes ECs to high concentrations of interstitial collagen I, which drives morphogenesis of new vessel sprouts. This abundant ECM protein supports VEGF-induced EC migration through β1 integrin signaling and Erk1/Erk2 activation, but it also drives directed cell migration in the absence of angiogenic factors [49]. Similarly, components of the provisional ECM, especially fibronectin, play a crucial role in this phase of angiogenic sprouting, and highly motile tip cells, for instance, show enrichment for β1 integrin, a fibronectin receptor [42]. These ECM proteins and their integrin receptors modulate EC migration by controlling cellular adhesion and by allowing functional connection between focal adhesions and actin cytoskeleton [50]. Ultimately, reorganization of the cytoskeleton enables ECs to complete their migration cycle through activation of small Rho GTPases, including CDC42, Rac1, and RhoA [51, 52]. However, the control of EC migration through cellular adhesion seems to be slightly more complex. Namely, fibronectin plays an additional role of orchestrating ECM matrix assembly by acting as a scaffold for a range of proteins produced by activated ECs [53]. The most intriguing subset includes matricellular proteins, which display unique effects on EC adhesion. When plated on tenascin-C and TSP-1, ECs do not undergo actin cytoskeleton remodeling and stress-fiber formation, although they engage integrin receptors and spread to a certain extent [54, 55]. In addition, soluble matricellular proteins induce focal adhesion restructuring and alterations in the stress fibers in strongly adherent cells without affecting integrin clustering and cell shape [56]. Such intermediate state of adhesion appears to favor cellular motility, enabling maximal migration [57]. In contrast, strong adhesion prevents the turnover of ECM-cellular contacts, and weak adhesion does not generate contractile force necessary for directed cellular migration [58]. Interestingly, although numerous members of the matricellular protein family, as well as some novel ECM-associated proteins such as EGFL7, appear to promote EC migration in such a manner, they all employ unique receptors as well as distinct signaling pathways to induce the state of intermediate adhesion [59, 60]. This strongly argues against their functional redundancy and suggests that their roles are highly contextual.
7.2.6 EC Proliferation and Tube Formation
While tip cells explore the surrounding environment and guide the sprout in the right direction, stalk cells actively proliferate and form lumens to extend and stabilize the sprout. Although the current model of vascular branching explains how the balance of Notch and Wnt signaling in the stalk cells maintains their active proliferation [61, 62], components of the ECM are indispensable for the regulation of both cell cycle and cell survival. In particular, the Ras-mitogen-activated protein (MAP) pathway seems to act as a master regulator of these cellular functions. For instance, fibronectin and vitronectin engage a subset of β1 integrins and αvβ3 integrin to activate Shc adaptor protein and Erk2 kinase, which in turn regulates cell-cycle progression [63]. In addition, integrins seem to cooperate with receptor tyrosine kinases (RTKs) to regulate the activity of cyclin-dependent kinases [64]. On the other hand, EC survival is largely dependent on cell adhesion and spreading, as well as activation of FAK and PI3K kinase signaling [65, 66]. Indeed, disruption of ECM-integrin interactions promotes apoptotic cell death [67]. In this context, matricellular proteins that maintain intermediate cell adhesion could be particularly important for the advancing vascular sprout as they would keep apoptosis at bay in migrating cells.
As previously mentioned, stalk cells following the invading tip cells eventually undergo complex molecular changes to form vascular lumens, although it still remains unclear whether this happens concurrently or subsequently to the sprout invasion [68, 69]. Numerous studies, using elegant in vitro and in vivo models, have demonstrated that this process requires a precise sequence of events and that it occurs through different mechanisms, depending on the size of the vessel or type of the vascular bed [70]. The first mechanism, also known as cell hollowing, has been demonstrated both in vitro and in vivo, and it involves formation of intracellular vacuoles that subsequently fuse and enable the cells to form continuous lumens [71, 72]. In contrast, cord hollowing occurs when lumen appears between the adjacent cells following complex junctional rearrangements, and changes in cell polarity and cell shape [73, 74]. However, a common feature of these different mechanisms is that they critically depend on numerous integrin–ECM interactions. For instance, in vitro studies have shown that collagen and fibrin/fibronectin matrices induce EC tubular morphogenesis through α2β1, α1β2, and αvβ3, α5β1 integrins, respectively [75, 76]. Indeed, inhibition or ablation of β1 integrin prevents lumen formation in chicken embryos [77] as well as mouse embryos through disruption of EC polarity [78]. These ECM–integrin interactions activate Src and FAK kinases as well as Rho GTPases to control intracellular vacuole formation and their coalescence [79, 80]. In contrast, ECM displays additional functions during cord hollowing. For example, it has recently been shown that a matricellular-like protein EGFL7 regulates EC adhesion and cell shape through activation of the RhoA pathway, enabling the formation of central lumen between the neighboring ECs [81, 82]. Finally, several recent reports revealed a surprising discovery that members of the vascular basement membrane do not only play a role in tube stabilization but also act earlier during the phase of lumen formation. Deletion of laminin γ1 in stem cells increases lumen diameter in angiogenic sprouts, and inhibition of basement membrane deposition in three-dimensional EC culture leads to lumen enlargement [83]. Interestingly, it seems that this is intricately connected with the sprout invasion and the tip cells themselves, as they show high expression of both nidogen-1 and nidogen-2, as well as laminin β1 [42].
7.2.7 Vessel Stabilization and Maturation
Finally, to end the angiogenic cycle and assume their resting phenotype, newly formed angiogenic branches recruit mural cells through activation of signaling pathways such as PDGF-(PDGF)-β, Ang1-Tie2, TGF-β, and SIP1-EDG1 [84]. One of the major functions that mural cells exert during this phase is production and deposition of vascular basement membrane matrix [85], which directly contributes to EC quiescence. For instance, both laminin and collagen XVIII knockout mice show increased angiogenic sprouting following application of angiogenic factors [86, 87], and these basement membrane proteins may directly supress EC proliferation and tube formation [88, 89]. To reinforce this process, a matricellular protein, CCN2, promotes pericyte recruitment by potentiating platelet-derived growth factor (PDGF) signaling; it also directs basement membrane assembly [90]. Another way through which basement membrane proteins stabilize angiogenic branches is the generation of matrikines. For instance, collagen XVIII undergoes partial proteolysis to form endostatin, which induces cell-cycle arrest and inhibits EC migration [91, 92]. Likewise, numerous fragments derived from collagen IV, including arresten, canstatin, and turmstatin, exert antiangiogenic activity through αv and β1 integrins, and through inhibition of matrix metalloproteinase (MMP) activity [19, 93]. Finally, concurrent with these dynamic changes in the extracellular environment, ECs and mural cells shift their gene expression program towards a more mature phenotype. ECs upregulate α3 and α6 integrin messenger RNA (mRNA) while pericytes dramatically increase the expression of α1, α3, and α6 integrin—receptors that preferentially bind laminins, nidogens, and collagen IV in the basement membrane [85]. In addition, activation of Hox genes that maintain EC quiescence (see the ‘Resting EC’ section) dramatically upregulate TSP-2, which inhibits EC adhesion, migration, survival, and tube formation [94, 95].
7.3 ECM and Tumor Vascularization
7.3.1 Introduction
One of the major steps during tumor growth and expansion is the development of a tumor vascular network, a process also known as angiogenic switch, which allows tumors to maintain their oxygen and nutrient supply and to remove the waste products [96]. Beyond such function, which is closely coupled with the blood supply, it has been well-documented that the angiogenic switch occurs at different stages of carcinogenesis, suggesting that tumor endothelium provides additional instructive cues necessary for tumor progression [97]. The main mechanism shown to contribute to tumor vascularization is angiogenic sprouting, which exploits much of the same molecular circuitry that drives physiological angiogenesis (see the ‘ECM and Blood Vessel Growth’ section). However, tumor-derived endothelium displays dramatic differences compared with its normal counterpart, reflected in irregular morphology, loss-of-function-specific properties, increased leakiness, defective basement membrane, and absence of close contact with mural cells [98]. This in turn causes activation of mechanisms that release angiogenic and permeability factors, which perpetuate the angiogenic cycle, maintaining the vessels in an immature state and continuously promoting the growth of new vessels [99].
7.3.2 Tumor Angiogenesis
A major component of reactive tumor stroma includes activated fibroblasts, which aberrantly deposit ECM proteins and enzymes, and therefore remodel local matrix topology and structure to form permissive grounds for tumor angiogenesis. Similar to what has been described in a physiological setting, ECM components regulate both initiation and maintenance of vessel growth. For instance, MMPs play a crucial role in releasing and activating matrix-bound proangiogenic factors [100] that initiate angiogenic sprouting. Indeed, mice lacking MMP-1 and MMP-9 show reduced tumor growth and angiogenesis [101, 102]. In addition, ECM ligands support all phases of vessel extension by regulating different functions of tumor ECs. The matricellular protein CCN1 promotes EC migration and aberrant neovascularization in pancreatic cancer [103]. EC survival during hypoxic conditions is mediated by a matrix-associated protein EGFL7 [104], while different collagens, which are excessively deposited within the stroma, may control EC proliferation through regulation of ECM stiffness [105, 106]. Finally, tenascin-C, which is highly expressed in tumor-derived endothelium [107], regulates multiple aspects of vascular sprouting by instructing EC migration, proliferation, and VEGF expression [108, 109] .
On the other hand, integrins have also been implicated in angiogenic sprouting during tumor development. Both αvβ3 and α5β1 integrin, which are strongly upregulated in activated endothelium, seem to be critical for this process; their antagonists effectively block tumor angiogenesis and induce tumor regression [110, 111]. In addition, collagen and laminin-binding receptors have been shown to play a role; function-directed antibodies against α1β1 and α2β1 integrin reduce tumor growth and angiogenesis [112]. Interestingly, however, genetic ablation models of these integrins in mice do not corroborate this data and the outcomes differ dramatically depending on the tumor type. Namely, mice lacking α2β1 integrin exhibit increased melanoma growth and angiogenesis, while in Lewis lung carcinoma these processes remain unchanged [113]. Furthermore, mice lacking β3 and β5 integrins show enhanced tumor growth and angiogenesis or, alternatively, they induce only transient inhibition of angiogenesis and tumor progression, without an effect on already-established tumors [114, 115]. This confirms that their role in tumor angiogenesis is highly context-dependent.
7.3.3 Additional Modes of Tumor Vascularization
Although angiogenic sprouting has been traditionally recognized as the main source of new vessels in developing tumors, extensive research within the last several years revealed unexpected and novel mechanisms of neovascularization. Not surprisingly, ECM plays a critical role in driving and facilitating these processes (Fig. 7.3) .

Fig. 7.3
Extracellular matrix (ECM) supports different modes of tumor vascularization. Similar to their role in physiological processes, ECM proteins promote angiogenic sprouting within tumors. However, recent findings uncovered alternative ways of how tumors increase their vascular supply and promote oncogenic signaling, and ECM components seem to be critical for these processes. The figure depicts some of these newly discovered modes of tumor vascularization
Endothelial Progenitor Cells
Contribution of endothelial progenitor cells (EPCs) to tumor vascularization has been intensively studied ever since they were isolated from the bone marrow [116] and peripheral blood [117], and shown to home into sites of active neovascularization. In sites such as tumors, there has been evidence that EPCs directly incorporate into already-existing vessels, although the extent of the contribution remains the subject of controversy [118]. Alternatively, EPCs appear to indirectly support tumor angiogenesis through paracrine mechanisms [119, 120]. Importantly, different components of the ECM promote and regulate each of the steps that EPCs need to complete to accomplish their function: mobilization, invasion of the tumor site, differentiation into mature ECs, and/or regulation of the pre-existing function of the ECs. For instance, integrins α4β1 and α4β7 are essential for mobilization of EPCs from the bone marrow microenvironment, while α6β1 integrin allows EPCs to respond to laminins, which act as a homing signal within the vascular basement membrane [121]. On the other hand, integrin α5β1 facilitates EPC homing to vascular injury sites where it binds fibronectin, which is abundantly present within the remodeling matrix [122, 123]. Additionally, EPCs adhere directly to the surface of the activated ECs by employing αvβ3 and αvβ5 integrins [124, 125]. During EPC differentiation into mature ECs, several ECM proteins play a crucial role, the most important of which is fibronectin. Namely, EPCs show higher adhesion and differentiation when plated on fibronectin compared with collagen, and they display continuous expression of fibronectin-binding integrins α4, α5, and αv [117, 126]. Fibronectin consequently promotes VEGF-induced EPC differentiation through ligation of α5β1 integrin [127]. In addition, a matricellular protein, CCN1, promotes EPC differentiation through regulation of negative transcriptional regulator Id1 [128]. Finally, in contrast to the process of differentiation, EPCs affect the angiogenic process through production of paracrine factors, which are dynamically regulated by different ECM substrates. For instance, gelatin, fibronectin, and fibrin proteolytic fragment E promote expression of VEGF, TGF-β1, stromal-cell derived factor (SDF)-1, and interleukin (IL)-8, which in turn facilitate EC tube formation and wound healing [129].
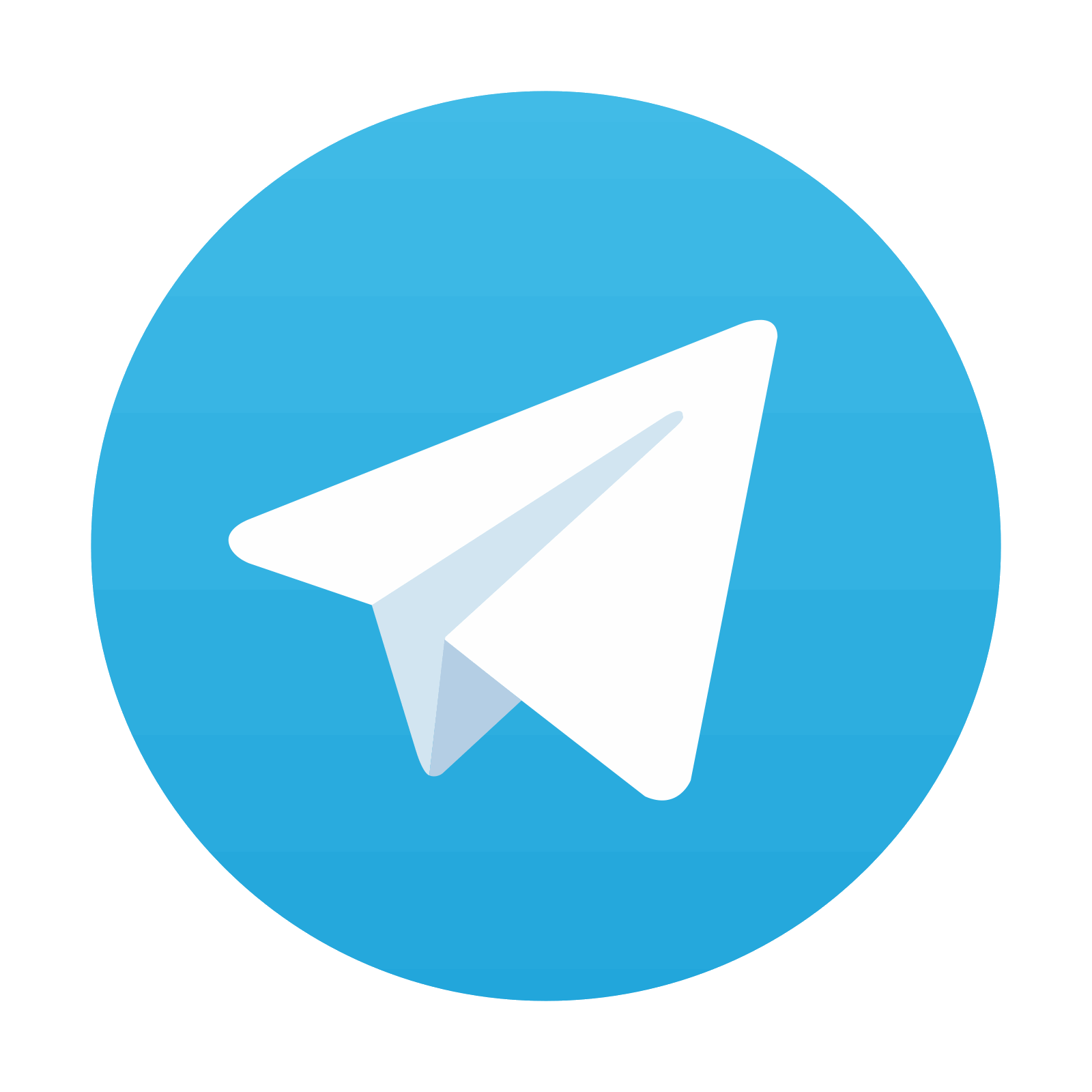
Stay updated, free articles. Join our Telegram channel
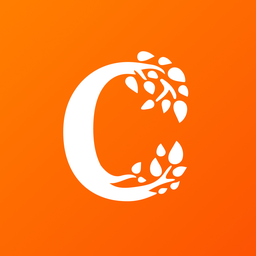
Full access? Get Clinical Tree
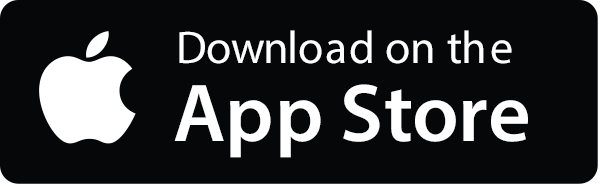
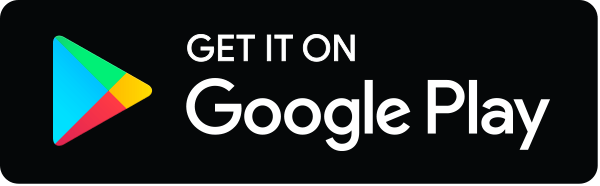