Clinical features of lung injury can vary from mild, self-limiting dyspnea to rapidly progressive and fatal respiratory failure. The clinical course of ALI can be generalized in 4 phases; however, not every patient passes through all these phases and the condition may resolve at any stage (Table 4.1). Extensive research continues to try to develop predictive biomarkers and genomic arrays for severity and resolution of lung injury; however, to date there are no useful clinical biomarkers or genomic readouts for prediction or goal directed therapy.
Consensus clinical definitions have been constructed to define lung injury and give a common vernacular among clinicians based on the pathophysiologic response to lung injury (Table 4.2). The definitions for ALI and ARDS are similar and include: a diffuse, bilateral disease process; pulmonary edema due to capillary leak (not hydrostatic edema from cardiogenic failure represented by a pulmonary capillary wedge pressure [PCWP] less than 18 cm H2O); and hypoxemia. The PCWP is rarely measured and is often inferred by exam or ECHO. ALI and ARDS only differ by the degree of hypoxemia (measured by a decrease in arterial oxygen tension: Pao2) and rising oxygen requirements (measured by an increase in the inspired fraction of oxygen: Fio2). This ratio is numerically expressed as the Pao2/Fio2 ratio (P/F ratio). The P/F ratio acts as a surrogate, quantitative data point to define worsening lung injury. A patient with a P/F ratio between 300 and 200, bilateral infiltrates on chest radiograph (CXR), and a PCWP less than 18 cm H2O by definition has ALI. As the P/F ratio falls below 200, a patient’s lung injury can be defined as ARDS. Unfortunately, the P/F ratio can be misleading in a mechanically ventilated patient, because it does not factor the level of mechanical support necessary for a patient to maintain an adequate Pao2 for a given Fio2. Therefore, physicians often discuss the degree of lung injury and progressive mechanical support in terms of oxygenation index (OI), which factors in the mean airway pressure (mPAW), the amount of supplemental inspired oxygen, and the resulting Pao2 (Figure 4.1). As with P/F ratios, the OI is a numerical representation attempting to quantify the degree of mechanical support and thus the degree of lung injury. OI, like P/F ratios, can be used to trend disease progression.
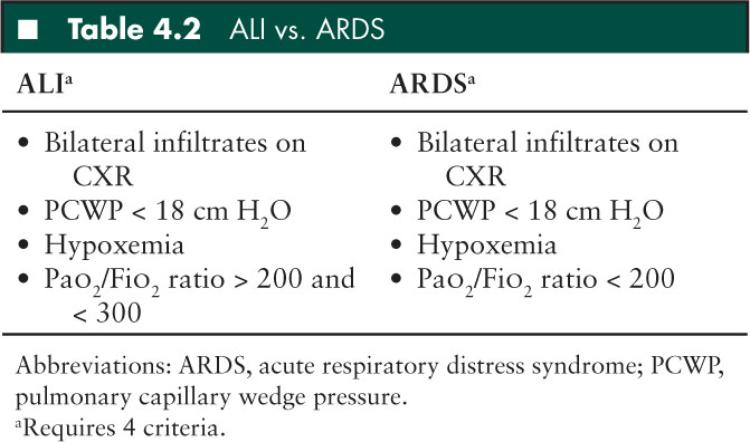
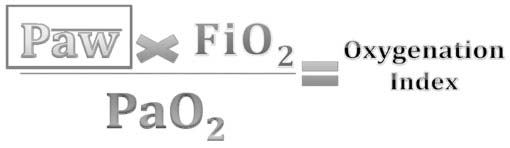
FIGURE 4.1 Formula to determine oxygenation index.
If lung injury can result from a varied group of disease processes, how can we use a broad, common set of definitions, such as ALI and ARDS, to define/discuss lung injury? In response to this question, Gattinoni and others have proposed that patients with ALI/ARDS should be considered as two separate groups (70) (Table 4.3). Pulmonary lung injury results from clinical conditions that cause direct lung injury (ie, pneumonia) whereas extrapulmonary lung injury follows an indirect mechanism of injury via systemic mediators of inflammation (ie, sepsis). In fact, despite meeting the same criteria for ARDS, the two subgroups of lung injury may differ with respect to pathologic mechanisms, appearances on CT, respiratory mechanics, and response to ventilatory strategies. However, despite diverse etiologies, the ultimate histologic appearances of lung injury are remarkably consistent. This ultimate, consistent histologic appearance, despite varied direct or indirect injury, allows ARDS to be considered a discrete clinical entity and this definition of ARDS has been used to study incidence and mortality.
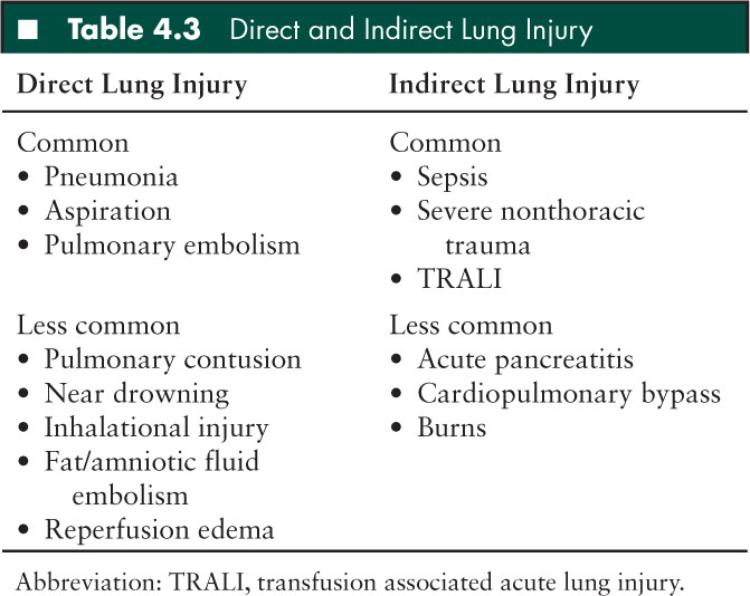
Principles of Lung Protective Strategies: Limiting Ventilator Associated Lung Injury
As lung injury progresses, in response to pulmonary or extrapulmonary injury, the lungs can be divided into three hypothetical regions: (1) Areas with severe collapse and alveolar flooding referred to as “dependent areas,” (2) recruitable areas with alveolar atelectasis referred to as “intermediate areas,” (3) normal lung referred to as “nondependent areas.” (Figure 4.2) The goal of mechanical ventilation is to recruit intermediate areas allowing improved gas exchange, spare normal areas of lung from ventilator associated lung injury (VALI), and give dependent collapsed regions of lung with alveolar flooding time to recover from the primary process (ie, pneumonia, sepsis). Lung recruitment of intermediate areas and prevention of VALI is accomplished by using peak end expiratory pressure (PEEP) and limiting tidal volume and plateau pressures. This can be a complicated task. Figure 4.3 provides a theoretical pressure-volume curve of the lung. As alveolar airway pressure increases there is an opening pressure (Pflex) required to overcome airway resistance and alveolar compliance (Compliance = DV/DP). Pressures below Pflex will lead to alveolar collapse, termed atelectasis. If airway pressure cycles above and below Pflex, alveoli will continually open and collapse, leading to wall shear stress and eventual damage referred to as atelectrauma. Following the hysteresis curve to the upper extent of the inspiratory limb, as pressure increases, there comes a point, termed Pmax, whereby the alveoli start to become overdistended. Above Pmax, shear stress again leads to alveolar damage referred to as volutrauma. Therefore, in theory, we attempt to keep tidal volumes on the most compliant part of the volume-pressure curve which is located above Pflex and below Pmax. This strategy is referred to as “open lung ventilation.” Triggered by the ARDSnet’s initial study, the use of low tidal volumes (6–8 mL/kg) with the addition of PEEP (open lung strategy) may reduce morbidity and mortality in patients with ARDS (Figure 4.4) (71). However, as lung injury to the normal and intermediate areas of the lungs progresses, the volume-pressure curve moves to the right as the compliance of the lungs decreases, leaving a smaller therapeutic window requiring an increase in PEEP, resulting in higher MAP to maintain recruitment of areas of normal and recruitable lung (Figure 4.5).
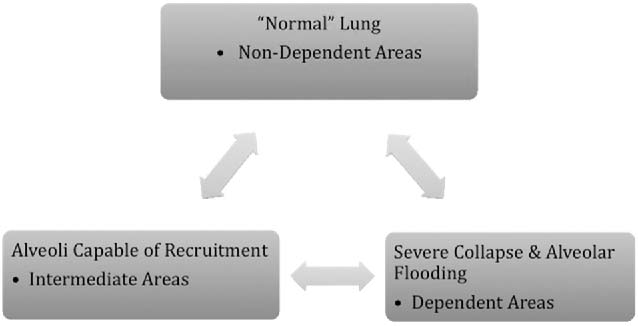
FIGURE 4.2 ALI/ARDS hypothetical lung sections.
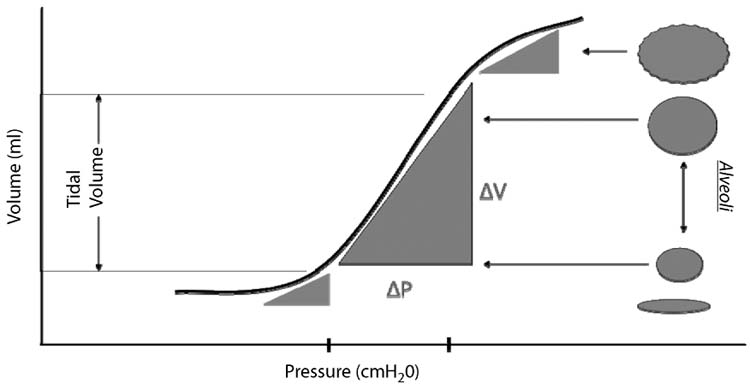
FIGURE 4.3 Volume-pressure curve.
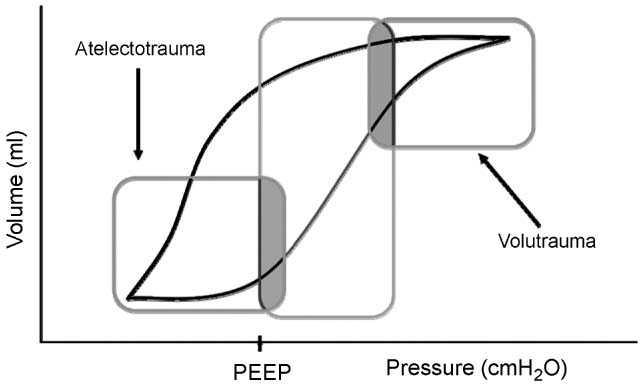
FIGURE 4.4 Lung protective ventilation.
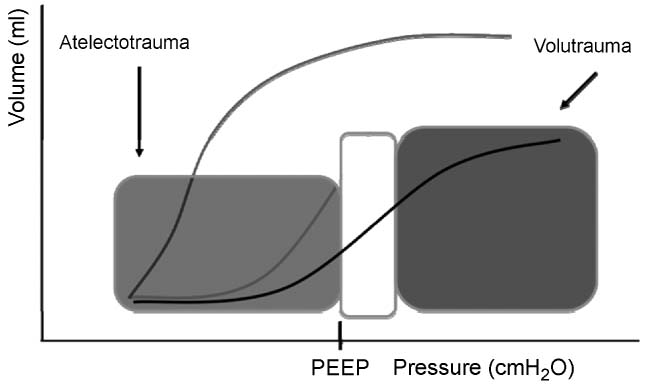
FIGURE 4.5 Lung protective ventilation.
Lung protective strategy attempts to decrease VALI by inhibiting: volutrauma, barotrauma, atelectrauma, oxygen toxicity, and biotrauma (Figure 4.6).
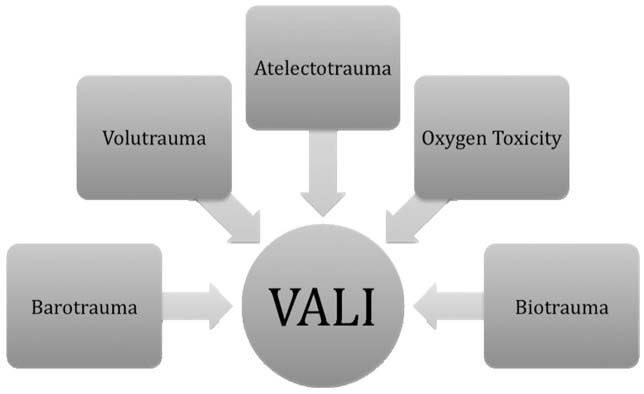
FIGURE 4.6 Factors leading to ventilator associated lung injury.
-
Low tidal volume: Despite using usual control groups in the ARDSnet original study, employing a low tidal volume approach of 6–8 mL/kg has become a standard of care.
-
PEEP: The advantages of PEEP as a distending pressure include increase in FRC, improvement of respiratory compliance, improvement of ventilation/perfusion mismatch, and redistribution of lung water/edema. PEEP ultimately improves arterial oxygenation. The use of low tidal volumes has been consistent across multiple recent trials, but the selection of PEEP in these trials has been highly variable. Animal models have shown that setting PEEP just above Pflex minimizes lung injury and inflammation (72). It can be difficult to determine the critical opening pressure of alveoli clinically. Therefore, most clinicians initially use a minimal distention strategy by setting PEEP between 5 and 9 cm H2O; as lung injury progresses and hypoxemia worsens, PEEP may be increased to increase mean plateau pressures (keep < 30–35 cm H2O) and aid in recruitment. These strategies have yet to show a decrease in mortality but have shown improvement in secondary outcomes (73). More precise methods of determining optimal PEEP in patients with ARDS include titration using dynamic compliance or static pressure-volume loops to identify the critical opening pressure. It is important to remember that as PEEP is increased and lung is recruited, intrathoracic pressure is also increased and may inhibit cardiac output by reducing venous return. Therefore, despite an improvement in oxygenation, oxygen delivery to vital organs may be compromised.
-
Plateau airway pressures: Sustained plateau airway pressures > 35 cm H2O can lead to barotraumas producing pneumothorax, pneumomediastinum, and subcutaneous emphysema. In an attempt to limit barotrauma, Paco2 is allowed to increase in the face of inadequate minute ventilation. This permissible hypercapnea can be employed as long as the patient tolerates the acidosis and is able to buffer with the renal retention of HCO3 and there are no contraindications from coexisting disease.
-
Partial ventilatory support: By avoiding neuromuscular blockade and paralysis of the muscles of the diaphragm, spontaneous ventilation can occur during mechanical ventilation. Partial ventilatory support can lead to improved alveolar recruitment and ventilation/perfusion (VQ) mismatch, improved venous return and cardiac output, and enhanced weaning from mechanical ventilation. Avoidance of neuromuscular blockade may also lead to decreased need for sedation.
■ BASIC MODES OF MECHANICAL VENTILATION
The most basic mode of mechanical ventilation is controlled mandatory ventilation (CMV) which delivers a set tidal volume at a set respiratory rate, thereby ensuring a constant minute-ventilation (Minute Ventilation = Tidal Volume · Respiratory Rate). CMV delivers a preset tidal volume at a preset rate regardless of the patient’s effort. Unlike CMV, intermittent mandatory ventilation (IMV) allows the patient to breath spontaneously between mandatory ventilator breaths. The most common type of IMV is synchronized IMV (SIMV), where mandatory ventilator breaths are synchronized via a timing window with the patient’s spontaneous breaths. SIMV has an assist window which is a period of time measured in seconds that is dependent on the set mandatory breaths. For example, if the set ventilator rate is 10 breaths per minute then the assist window is 6 seconds. During the assist window, if the ventilator does not sense a spontaneous breath from the patient, the ventilator will deliver a mandatory breath. SIMV is commonly combined with pressure support (PS): SIMV + PS. When a ventilator is placed into SIMV + PS, the machine senses the patient initiating a breath and a preset positive pressure is delivered during the patient’s breath in an attempt to support an adequate tidal volume and, thus, minute ventilation. PS is often set between 5 and 20 cm H2O. SIMV + PS is advantageous because it allows for partial ventilatory support which improves cardiopulmonary mechanics and improves a patient’s ability to wean from mechanical support. The disadvantage of SIMV + PS is that it does not assure minute ventilation as the mechanical breaths are decreased and the patient becomes less dependent on mandatory breaths to maintain minute ventilation. If the patient is not ready for decreased support the work of breathing may increase and ventilation will be impaired.
Once the mode of ventilation is set (CMV, IMV, SIMV, SIMV + PS) the clinician must determine the type of ventilator breath delivered to the patient. The most common types of breaths delivered are either pressure controlled (PC) or volume controlled (VC) and are referred to as SIMV-PC + PS or SIMV-VC + PS, respectively.
-
Pressure-controlled ventilation: PC ventilation delivers a pressure limited breath at a preset respiratory rate and inspiratory time (Ti). Tidal volume is a dependent variable during PC ventilation, and is determined by the preset pressure limit and the compliance and resistance of the respiratory system. If the respiratory systems compliance decreases or airway resistance increases, the preset pressure limit may not be adequate to maintain a tidal volume necessary to support a patient’s minute ventilation. The flow waveform during PC ventilation is a decelerating pattern that follows a pressure gradient between the pressure delivered and alveolar pressure. Gas flows into airways along this pressure gradient, and as the alveolar volume increases and the pressure gradient between delivered breath and alveolar pressure narrows, gas flow will slow. When the clinician sets the Ti, gas flow maintains a decelerating pattern and even after the gas flow has stopped, MAP is maintained for the length of the set Ti. This combination of a high initial flow with a decelerating flow pattern and maintained set airway pressure over set Ti, may improve aeration by recruiting stiff, noncompliant sections of lung in ALI and ARDS. PC may also be useful in patients with air leak, either via a fistula or around the endotracheal tube, by maintaining gas flow until the set pressure is obtained and tidal volume achieved.
-
Volume-controlled ventilation: VC ventilation delivers a preset tidal volume breath during a preset Ti and at a preset respiratory rate. By setting volume control, alveolar overdistention may be avoided, limiting ventilator induced lung injury (volutrauma). During VC ventilation, airway and alveolar pressures are dependent variables, and as lung injury worsens mandatory tidal volumes may be maintained despite rising high peak inspiratory pressures to guarantee minute ventilation. These rising peak airway pressures may also lead to ventilator induced lung injury. Gas flow is constant during Ti. VC may be advantageous in patients with changing airway compliance, that is, intraoperative chest or abdominal surgery, so tidal volume may be consistent.
■ ADVANCED MODES OF MECHANICAL VENTILATION
Conventional mechanical ventilation fails due to inadequate CO2 clearance (despite permissible hypercapnea, usually with persistent acidosis), poor oxygen delivery, exacerbation of lung injury beyond limits of protective ventilation, and inhibition of cardiovascular function due to increasing intrathoracic pressure. Traditionally, high frequency oscillatory ventilation (HFOV) and airway pressure release ventilation (APRV) have evolved as alternatives to failed conventional ventilation; however, APRV and HFOV may be used as a primary mode of ventilation in certain situations such as with air leak syndrome or postpneumonectomy. Both modes have the advantage of ventilating patients at full lung volumes on the expiratory limb of the pressure-volume curve while attempting to avoid phasic changes.
-
High frequency oscillatory ventilation: HFOV has become the mainstay of mechanical ventilation in pediatric patients with evolving ALI that is unresponse to conventional modes of ventilation. In fact, some authors have suggested that early institution of HFOV during ALI may improve survival by providing open lung ventilation without overdistention and further VALI (74). Therefore, HFOV allows for optimal alveolar recruitment with lower airway pressures, while minimizing atelectrauma associated with phasic alveolar opening and closing. A piston/diaphragm creates gas flow through by three methods: bulk axial flow, interregional gas mixing (pendelluft), and molecular diffusion. Tidal volumes are dependent on the patient’s compliance, endotracheal tube size, device frequency, and device amplitude. Tidal volume is inversely related to cyclic frequency: VCO2 > Frequency × VT2. Transitioning from conventional modes of ventilation to HFOV, the initial power setting (ΔP/amplitude) is adjusted to visible chest “wiggle” from the clavicles to the abdomen or pelvis. Mean airway pressures (mPAW) is initially set approximately 5 cm H2O greater than the last mPAW on conventional ventilation just prior to the initiation of HFOV. Traditionally, tidal volumes in HFOV are considered to be just above FRC; however, it is difficult to measure actual tidal volumes and provide precise “optimal” lung volume strategy. Clinically, mean airway pressure (mPAW) is titrated by upward by 1 to 2 cm H2O till oxygenation improves and inspired oxygen concentrations can be weaned to less toxic levels (below 0.60). While titrating mPAW it is important to assess for overdistention by following chest radiographs. Overdistention/hyperinflation is determined by greater than 9 posterior ribs or flattened hemidiaphragms on chest x-ray. Table 4.4 lists initial frequency settings.
-
HFOV is the only mode of ventilation with active expiration. If hypercarbia, despite allowances for permissible hypercapnea, leads to profound respiratory acidosis and patient instability, then minute ventilation can be improved by several means during HFOV. First, one of the drawbacks of HFOV is the lack of spontaneous ventilation and adequate airway clearance, therefore inline suction (without breaking into the circuit causing derecruitment) should be used to ensure adequate airway and endotracheal tube patency and lung recruitment. Second, ΔP should be increased to maximize lung recruitment and increase minute ventilation. Third, frequency (Hz) can be slowly decreased to enhance lung recruitment and increase minute ventilation. Finally, the endotracheal tube cuff should be deflated to allow additional escape of CO2 around the endotracheal tube.
-
Disadvantages of HFOV include lack of partial ventilatory support leading to increased sedation and paralysis requirements, cardiopulmonary interactions due to higher mPAW and decreased venous return, and loss of alveolar recruitment if circuit detached for suctioning or manual ventilation.
-
Airway pressure release ventilation: APRV is essentially continuous positive airway pressure (CPAP) with brief, intermittent release coupled with spontaneous ventilation. The high CPAP level (Phigh) maintains alveolar recruitment and aids in oxygenation over a period of time (Thigh), and the timed release to a low pressure (Plow) minimizes resistance to expiratory flow and carbon dioxide removal. In addition, the patient is able to spontaneous breath during all phases, Phigh and Plow, potentially allowing for improved pulmonary mechanics and gas exchange. APRV differs from other modes of ventilation because it relies on an intermittent decrease in airway pressure, instead of an increase in airway pressure to maintain an open lung strategy for ventilation. Therefore, the release time (Tlow) should be set long enough to allow for an adequate tidal volume (6–8 mL/kg), but short enough to avoid alveolar collapse and atelectrauma.
-
In summary, the operator-controlled parameters in APRV are: Phigh, Thigh, Plow, Tlow, and Fio2. Recommendations for implementing APRV are limited in pediatrics, and thus extrapolated from adult recommendations (75). Plow is initially set a zero. Phigh can be set by several methods such as plateau pressures or 75% of peak inspiratory pressure; however, when transitioning from conventional modes of ventilation Phigh is often determined by mPAW pressure formula (see Table 4.5) where the mPAW is set 2 to 3 cm H2O above conventional mPAW. To determine Thigh and Tlow, first determine the total cycle time according to a normal respiratory rate range for the patient’s age (ie, a respiratory rate of 20 yields a total cycle time of 3 seconds). Thigh will be the total cycle time minus a Tlow of 0.2 to 0.6 seconds, initially starting at 0.4 seconds (ie, Total cycle time of 3 seconds yields a Thigh of 2.6 seconds and Tlow of 0.4 seconds). Transitioning to APRV, like transitioning to HFOV, will take time for optimal lung recruitment. After several hours if patient continues to have severe hypoxemia, Thigh can be increased to aid in oxygenation. Once established, Plow and Tlow usually do not require further changes; however, as lung compliance improves, Phigh and Thigh can be decreased and increased respectively to wean a patient toward a target of a continual CPAP of 5 to 6 cm H2O in preparation for extubation.
-
APRV may be advantageous to other modes of advanced mechanical ventilation because of the patient’s ability to breath spontaneously throughout the entire ventilatory cycle. This improves respiratory mechanics and reduces the need for sedation and neuromuscular blockade.
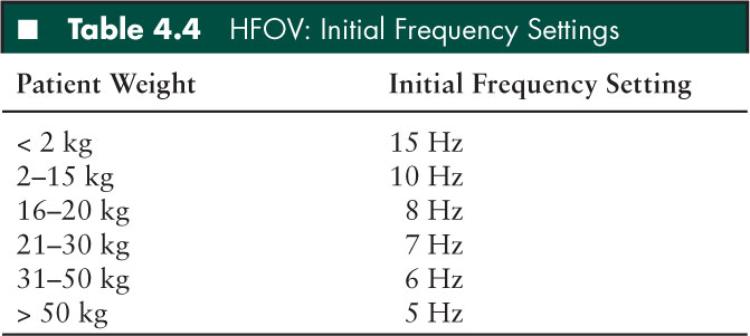
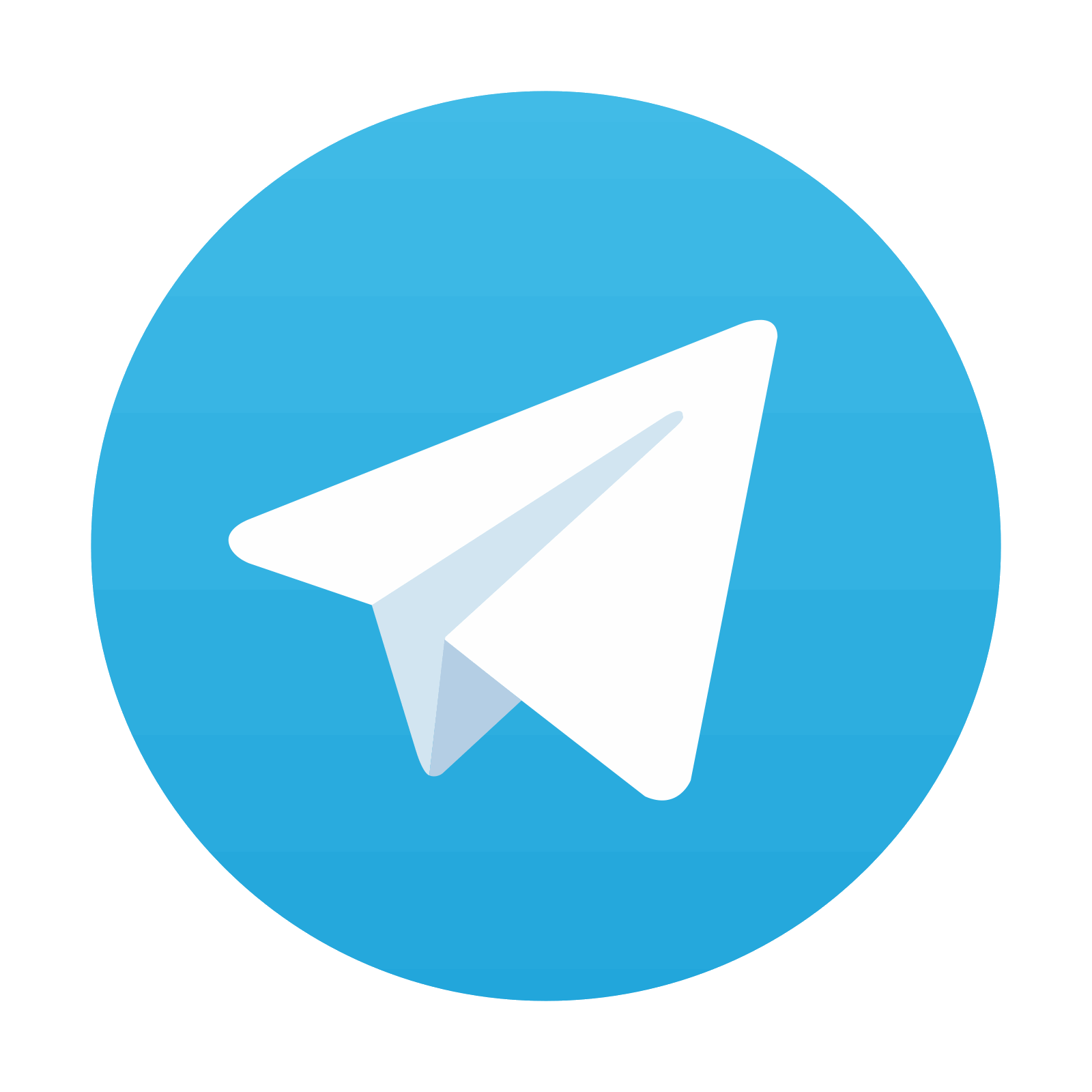
Stay updated, free articles. Join our Telegram channel
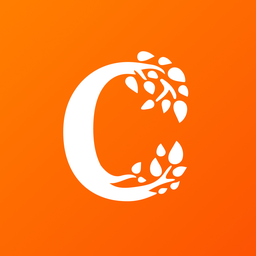
Full access? Get Clinical Tree
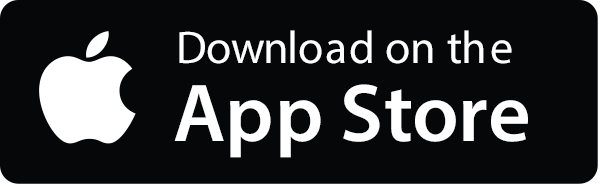
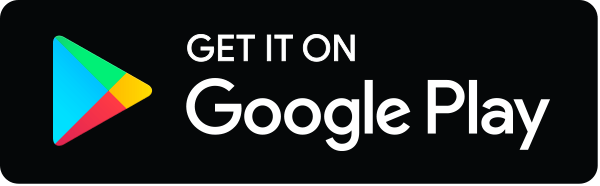