Fig. 4.1
(a) An example of a reciprocal translocation involving chromosomes 16 and 22. The carrier parent can transmit an unbalanced translocation to the fetus. (b) An example of a Robertsonian translocation involving chromosome 13
Robertsonian translocation (ROB) is a form of chromosomal rearrangement that involves one of the short-arm five acrocentric chromosome pairs, namely 13, 14, 15, 21, and 22 [11, 12]. They are named after the American biologist William Rees Brebner Robertson (1881–1941), who first described a Robertsonian translocation in grasshoppers in 1916 [13] (Fig. 4.1b).
Robertsonian translocations result in a loss of part or all of the short arms and fusion of the long arms of the chromosome involved keeping one or two centromeres in between. The short arms of these chromosomes (also called satellites) are lost but since they contain redundant DNA their loss does not cause harm. A Robertsonian translocation in balanced form causes no health issues. In unbalanced forms, Robertsonian translocations can result in trisomies or monosomies. While some trisomies may survive (21, 18, or 13), at least through pregnancy all autosomal monosomies are lethal and will usually result in a first-trimester miscarriage.
Inversions
Inversion is caused by two breaks occurring in the same chromosome with the intervening genetic material inverted before the breaks are repaired. Although, in a balanced situation, no genetic material is lost or duplicated, the rearrangement may alter gene function, if the break is in a gene or its promoter. The inversion can be paracentric, when the two breaks are in one of the arms with a 180° rotation of the segment involved or pericentric if the breaks are in each of the chromosome arms with a 180° rotation around the centromere [14, 15].
Carriers of a paracentric inversion make either balanced gametes or gametes with acentric and dicentric gametes, the products of which are usually lethal. Thus the risk of abnormal offspring is extremely low. Carriers of a pericentric inversions can form unbalanced gametes with duplication and deletion of the segment involved and is thus at high risk to produce abnormal gametes and thus abnormal offspring.
Techniques and Methods
FISH Analysis
This technique provides a rapid method for identifying a microscopic or submicroscopic deletion and/or duplication of a segment of a chromosome or a whole chromosome. In this technique a segment of a chromosome called a probe is being stained with a fluorescent dye and is being allowed to hybridize to the karyotype. The probe will hybridize to its corresponding segment on the karyotype which is being tested. The karyotype in an interphase or a metaphase state is being checked with a fluorescent microscope to see if the segment is deleted or duplicated [16, 17].
Chromosomal Micro Array Analysis
Chromosomal micro array (CMA ) is designed and utilized to detect copy number variants (CNVs), i.e., deletions or duplications (and in some platforms, loss of heterozygosity (LOH) and thus uniparental disomy (UPD)).
CNVs consist of up to 12 % of the human genome. Many of the CNVs are considered polymorphic and/or familial and these are usually nonpathogenic. However, many are pathogenic and thus associated with abnormalities/mental retardation (MR) or predisposition to abnormalities/MR. CMA has a substantially higher resolution than microscopic chromosome abnormality and can thus identify submicroscopic deletion/duplication which cannot be identified by the traditional chromosome analysis.
The evolution of CMAs was rapid and directed toward new clinical targets and fields. Genomic PCR products, bacterial artificial chromosomes (BACs), and oligonucleotides all were used in comparative genomic hybridization CGH analysis (a type of CMA) [18–20]. While the validity and use of CMA were emerging, array designs were addressing the question of coverage and resolution. Probe coverage on chromosomal backbone is variable and needed for method validation and accuracy. Disease-targeted probes were located according to known loci and interpretable regions. The use of such a high-resolution/high-coverage arrays contributed significantly to the detection discovery of new copy variants related to clinical conditions and to the delineation of many others.
Single-nucleotide polymorphism (SNP) array is a type of CMA which uses polymorphisms within a population. There are around 50 million SNPs that have been identified in the human genome [21]. An SNP array is a useful tool for studying variations between whole genomes. SNP arrays can be used for determining disease susceptibility, measuring the efficacy of drug therapies, etc. An SNP array is also being used to determine CNVs (submicroscopic deletions and duplications). A significant advantage of SNP array over CGH array is the ability to report on regions of LOH and thus identify cases with UPD, consanguinity, and products of incest relationship. A significant drawback of all CMAs is the inability to detect balanced changes such as balanced translocation (reciprocal or Robertsonian).
Next-Generation Sequencing and WES/ WGS
The polymerase chain reaction (PCR) is a technology used to amplify a single copy or a few copies of a piece of DNA across several orders of magnitude, generating thousands to millions of copies of a particular DNA sequence [22].
DNA sequencing is the process of determining the precise order of nucleotides within a DNA molecule. The first DNA sequences were obtained in the early 1970s. While at first the task was extremely time consuming and laborious, development of fluorescence-based sequencing methods with automated analysis has made DNA sequencing [23] easier and substantially faster [24].
Several new methods for DNA sequencing were developed in the mid to late 1990s and were implemented in commercial DNA sequencers almost a decade later.
Basically, all the methods use a random surface-PCR arraying method coupled to “base-by-base” sequencing method. Later, several commercial companies begun to market “massively parallel signature sequencing,” or MPSS. This method incorporated a parallelized, adapter/ligation-mediated, bead-based sequencing technology and served as the first commercially available “next-generation” sequencing (NGS) method [25]. Sharply reduced costs and increased availability began a new era for massive sequencing, and genome-wide association studies (GWAS) emerged. Dealing with common diseases, GWAS’ approach was to seek genomic variants “that will be able to assist in to mapping risk groups.” In the investigation of single-gene disorders “whole-genome or whole-exome sequencing” facilitated the detection of new genes and expanded the spectrum of many known genetic customized NGS panels were developed, by which a parallel sequencing of several genes that may be involved in a common phenotype is being carried out. This approach is accurate and a relatively inexpensive option for identifying a causative gene in diseases with known genetic etiology.
MicroRNA Analysis
MicroRNAs constitute a recently discovered class of noncoding RNAs that play key roles in the regulation of gene expression [26–29]. Acting at the posttranscriptional level, these small molecules fine-tune the expression of up to 30 % of all mammalian protein-encoding genes. Mature microRNAs are short, single-stranded RNA molecules approximately 22 nucleotides in length. MicroRNAs are encoded by multiple loci, some of which are organized in tandemly co-transcribed clusters. MicroRNA genes are transcribed by RNA polymerase II as large primary transcripts (pri-microRNA) that are processed by a protein complex containing the RNase III enzyme Drosha, to form an approximately 70-nucleotide precursor microRNA (pre-microRNA). This precursor is subsequently transported to the cytoplasm where it is processed by a second RNase III enzyme, Dicer, to form a mature microRNA of approximately 22 nucleotides. The mature microRNA is then incorporated into a ribonuclear particle to form the RNA-induced silencing complex, RISC, which mediates gene silencing. MicroRNAs usually induce gene silencing by binding to target sites found within the 3′UTR of the targeted mRNA. This interaction prevents protein production by suppressing protein synthesis and/or by initiating mRNA degradation. Since most target sites on the mRNA have only partial base complementarity with their corresponding microRNA, individual microRNAs may target as many as 100 different mRNAs. Moreover, individual mRNAs may contain multiple binding sites for different microRNAs, resulting in a complex regulatory network. MicroRNAs have been shown to be involved in a wide range of biological processes such as cell cycle control, apoptosis, and several developmental and physiological processes including stem cell differentiation, hematopoiesis, hypoxia, cardiac and skeletal muscle development, neurogenesis, insulin secretion, cholesterol metabolism, aging, immune responses, and viral replication. In addition, highly tissue-specific expression and distinct temporal expression patterns during embryogenesis suggest that microRNAs play a key role in the differentiation and maintenance of tissue identity. In addition to their important roles in healthy individuals, microRNAs have also been implicated in a number of diseases including a broad range of cancers, heart disease, and neurological disorders. Consequently, microRNAs are intensely studied as candidates for diagnostic and prognostic biomarkers and predictors of drug response.
Genetic Basis of Miscarriage
Prospective cohort studies demonstrated that only around one-third of conceptions progress to a live birth [30, 31]. The incidence of early clinical pregnancy loss is estimated to be 15 %, and is (mainly maternal) age dependent; late losses between 12 and 22 weeks occur less frequently and constitute around 4 % [5].
The prevalence of RPL is much lower and the reported prevalence varies by inclusion criteria. If only clinical pregnancies are included, the prevalence is 0.8–1.4 %, while if preclinical pregnancies are included as well the prevalence can be high as 3 % [32]. As defined by The Practice Committee of the American Society for Reproductive Medicine, RPL is etiologically a heterogeneous condition. In the literature, a multifactorial background of RPL is accepted at the population level, but at the individual level of a specific couple, RPL is considered to be monofactorial. However, none of the reported causes exhibit high sensitivity or specificity regarding RPL, meaning etiologic causes of RPL may occur in couples with RPL as well as in couples with normal fecundity [33].
In about 50 % of the cases, miscarriage is the result of chromosome abnormalities. About 50 % of these are the result of trisomies and 50 % are non-trisomies, mainly monosomy X and triploidy. Thus, most cases are not inherited and caused by de novo numerical chromosome aberrations (monosomies, polyploidies, and trisomies) originating in the gametes prior to fertilization or occurring after fertilization. Usually those tend to be nonrecurring abnormalities with some associated with maternal age. Less frequently the miscarriage is a result of a gamete with an unbalanced chromosomal translocation inherited from a parent carrying a balanced chromosome rearrangement.
Recessive genes or interactions of several genes may also cause lethal malformations in the fetus/embryo; however the incidence of miscarriages caused by genes with an autosomal recessive mode of inheritance is uncertain and probably low.
Fetal Aneuploidy
In the general population, the risk of miscarriage due to fetal aneuploidy increases with maternal age. The risk of trisomy is known to increase with maternal age, but translocations do not seem to be age related.
This age-related risk of miscarriage also applies to RPL [34]. Most miscarriages with aneuploidies are de novo, with a recurrence risk being low, and the risk is being determined by the mother’s age-related risk. In fact, several reports demonstrated that the recurrence risk of a miscarriage seems to be higher following a miscarriage of a chromosomally normal conceptus than after a chromosomally abnormal miscarriage [32, 35].
In a series of 167 cases Carp et al. [32] described the chromosomal abnormalities in 36 cases of RPL and showed that the most common chromosomal abnormality was trisomy, found in 24 of the 36 chromosomally abnormal embryos. Trisomy 21 was the most common aberration, appearing in 5 of the 24 trisomies followed by trisomies 16 and 18, triploidy, monosomy X, and unbalanced translocations. The authors concluded that karyotyping the abortuses allows the patient to be given a more accurate diagnosis and more useful prognostic information regarding subsequent pregnancy outcomes. A patient who miscarried an aneuploid embryo had a better chance for a subsequent live birth than the patient with a euploid miscarriage (OR = 3.11). This finding was also found by Ogasawara et al. [36] and was statistically significant. Warburton et al. [37] reported on 273 women who had two abortuses karyotyped. They concluded that there is no increased risk of trisomy after a previous trisomic miscarriage, and that the prognosis is favorable for these patients. This may not be the case in euploid miscarriages. Carp et al. [32] reported that all 11 patients with a euploid miscarriage who had a repeat karyotypically normal loss had a higher recurrence with another euploid fetus, suggesting an alternative cause of miscarriage. Philipp et al. [38] explored in several studies the correlation between embryonic disorganization detected by embryoscopy and chromosome abnormalities. In their report from 2003, fetal malformations were observed in 85 % of cases presenting with early clinical miscarriage. The same study also demonstrated that 75 % of the fetuses had an abnormal karyotype. Only a small proportion of fetuses with chromosomal aberration can survive to term. Even trisomy 21, the most common trisomy observed in neonates at term, has demise in 80 % in utero or in the neonatal period [35, 39]. Ven der Berg [40] summarized six studies [34, 36, 38, 41–45] describing cytogenetic abnormalities in RPL. Of the 1359 successfully karyotyped miscarriage samples, 39 % had an abnormal karyotype. The spectrum of chromosome abnormalities included 90 % numerical abnormalities, 3 % structural abnormalities, and 13 % other chromosome abnormalities (mosaicism, double, triple, and quadruple trisomies, and autosomal monosomy; some samples had more than one abnormality). Most cases of chromosomal abnormalities are due to maternal non-disjunction of Meiosis I.
Balanced parental rearrangements are found in 3–6 % of RPL cases [8] and the most commonly encountered parental chromosome rearrangements include balanced translocations and inversions. This risk of miscarriage in these cases is influenced by the size and the genetic content of the rearranged chromosomal segments.
Cryptic CNVs
Some of the miscarriages may be due to submicroscopic chromosomal changes [38]. The introduction of CMA enabled a search for CNVs associated with RPL.
Since CMA does not require cell culture, it provides us with more accurate information regarding the incidence and type of chromosome abnormalities associated with miscarriages. Rosenfeld et al. [46] showed that using CMA and excluding cases referred with known microscopic abnormal karyotypes, clinically significant abnormalities were identified in 12.8 % (64/499) of the miscarriages/stillborn. Detection rates were significantly higher with earlier gestational age and clinically significant abnormalities were identified in 6.9 % (20/288) of cases with normal karyotypes. This detection rate did not significantly vary with gestational age, suggesting that, unlike aneuploidy, the contribution of submicroscopic chromosome abnormalities to fetal demise does not vary with gestational age. SNP analysis detected abnormalities of potential clinical significance, including female triploidy, in an additional 6.5 % (7/107).
Different reports affirmed the value of CMA in cases where obtaining tissue for karyotype was difficult or unavailable (e.g., culture failure) [47–49].
As published by Reddy (as a part of the Stillbirth Collaborative Research Network), of the 51 samples with pathologic CNVs, only 43.1 % (n 22) were detected by karyotype [50]. Only a few reports regarding the use of CMA in recurrent pregnancy loss could be identified. Although the detection rates using CMA are higher than in karyotype the interpretation of the findings is more complex especially when inherited [51].
The literature regarding CNVs in sporadic miscarriage as well as RPL is sparse. van der Berg [40] summarized data from seven studies reporting findings of CMA for detecting submicroscopic genetic abnormalities in sporadic miscarriage samples; those studies refer to 362 miscarriages in total. These combined studies suggest that in 5 % of all sporadic miscarriages CNVs are found that cannot be detected by conventional cytogenetic analysis. In their report, Warren et al. reported CMA results in one-third (8/25) of their cohort presenting with RPL. Four de novo submicroscopic chromosome abnormalities were found by two different arrays (Xp22.31, 12q33.3, 5p15.33, and Xp22.31); three were duplications and one deletion. None of the CNVs seem to have gene content reported that correlates to pregnancy loss [49].
The clinical relevance of these CNVs is still being learned and no conclusive findings are yet available. Issues like inherited versus de novo, size and location, variable expressivity and penetrance, and parental origin are all important aspects of CNV interpretation. A small number of cases are reported with parental testing, allowing only limited conclusions to be drawn [51, 52]. Further studies are needed to determine the size and distribution of the de novo CNVs in this group and whether these CNVs have an etiologic role in the miscarriages.
Role of MiRNAs in Miscarriage
MicroRNA (miRNA ) has a function in posttranscriptional regulation of gene expression by targeting mRNAs for degradation and/or translational repression [53], and thus has a role in the repression of protein expression. Thus there might be a role for miRNA in RPL.
In patients with recurrent implantation failure some miRNAs were differently expressed. The affected pathways are of Wnt signaling and cell cycle and formation of adhesion molecules [54]. Hu et al. [55] reported two single-nucleotide polymorphisms (SNPs) within the pri-miR-125a in 217 Han Chinese patients with RPL compared with 431 controls. This SNP is downregulated in pre- and mature-miR-125a, leading to reduction in miR-125a and to less efficient inhibition of target genes, LIFR and ERBB2, which play important roles in the embryo implantation and decidualization. Other miRNAs associated with RPL were reported by Jeon et al. [56] in their study; miR-196a2CC, miR-499AG+GG, and the miR-196a2CC/miR-499AG+GG combination was associated with increased risk of idiopathic RPL, indicating that the functions of those miRNAs and their target genes may be important in the etiology of RPL.
The assumption that the miRNA can profile to explain or predict RPL (or an ongoing pregnancy) is appealing, but more studies are required to consolidate the data.
The Genetic Work-Up of RPL
Parental
Traditionally, chromosome analysis has been recommended for couples with RPL although some controversy is still surrounding this recommendation. Those in favor of routine chromosome analysis suggest that it should be included in the counseling provided to couples with RPL regarding the recurrence risk and the chance of having a fetus/newborn with unbalanced chromosomal rearrangement. Those who oppose offering routine karyotyping for couples with RPL refer to a study pointing out that carrier couples with at least two previous miscarriages had the same chance of having a healthy child as non-carrier couples with at least two miscarriages (83 % and 84 %, respectively), and more importantly a low risk (0.8 %) of pregnancies with an unbalanced karyotype surviving into the second trimester [57].
However current clinical guidelines do recommend parental karyotyping as part of the evaluation of couples with RPL, especially if the maternal age is low at the second miscarriage, or if there is a history of two or more miscarriages in first-degree relatives.
De Jong et al. [58] stated that knowing the result of the fetal karyotype does not predict anything for the next pregnancy, since usually the value of this knowledge is in explaining to the parents the reason for the specific miscarriage in case of an abnormal fetal karyotype. There may be another advantage of such knowledge. In their original paper, Mevorach-Zussman et al. [59] stated that anxiety is a major component in RPL couples. In their cohort supportive care was not sufficient, so may be solid medical knowledge can actually contribute to relieving the anxiety of RPL couples.
Routine chromosomal microarray for couples with RPL is controversial and should be based on the results of CMA done on the product of conception. Couples with RPL due to inherited chromosome abnormality will most probably have balanced chromosome rearrangement which cannot be detected by CMA.
miRNA analysis has not been recommended in the routine investigation recommended to couples with RPL. As in many other medical fields, miRNA seems to be a promising direction for the developing medications targeting the regulation of gene expression.
Aiming to improve pregnancy outcomes, and achieving a healthy newborn, assisted reproductive technologies (ARTs) have been used for couples with RPL. Preimplantation genetic diagnosis (PGD ) can be used to target on known chromosomal aberrations or preimplantation genetic screening used to screen for chromosomal aberrations (PGS) [60]. Both methods select the best suitable (without chromosomal abnormality) fertilized egg to be transferred to the uterus.
The second possibility is assuming that the etiology lies within the embryonic environment, aka the uterus, and thus placing the embryo in a surrogate uterus.
PGS is CMA done on a cell obtained from the blastocyst. PGS can only be performed in conjunction with an in vitro fertilization (IVF). In contrast, PGD is done to evaluate the embryos for a single-gene disorder carried by the couple, such as sickle cell disease or cystic fibrosis, where carrier status has been documented in each of the parents [61].
In high-risk patients for an aneuploid embryo, such as advanced maternal age, recurrent miscarriage, repeated implantation failure, and severe male factor patients [62–64], PGS was suggested as a method to improve pregnancy success rate. Because the majority of data was collected by karyotyping of products of conception, many PGS cycles were focused on common aneuploidies, mainly utilizing fluorescence in situ hybridization (FISH) approaches. Unfortunately, results from this approach were disappointing. Mastenbroek et al. [65] concluded that PGS significantly reduced the rates of pregnancies and live births following IVF in women with advanced maternal age. A renewed interest in PGS was noted with the introduction of microarray technologies. To date the data from studies is limited by the methodology limitations and the small number of patients included in these studies and only a few randomized controlled trials addressing the question of whether or not PGS should be used in RPL patients [64] are available.
Several studies have shown that PGD for familial translocations reduced miscarriage rates from >90 to <15 % [66–69]. The same reduction rates were noted in RPL patients [70].
Christiansen [71] referred to available studies regarding PGS in PRL cases. Comparing four observational studies on RPL to seven studies on natural conception, live birth rates were 35 % and 41 %, respectively; miscarriage rate for the PGS group was 9 %, and 28 % in the natural conception group. It was suggested that since conception after PGS is expected to take longer than natural conception, the most appropriate way to compare pregnancy outcome after PGS versus natural conception is to register the live birth rate per time unit.
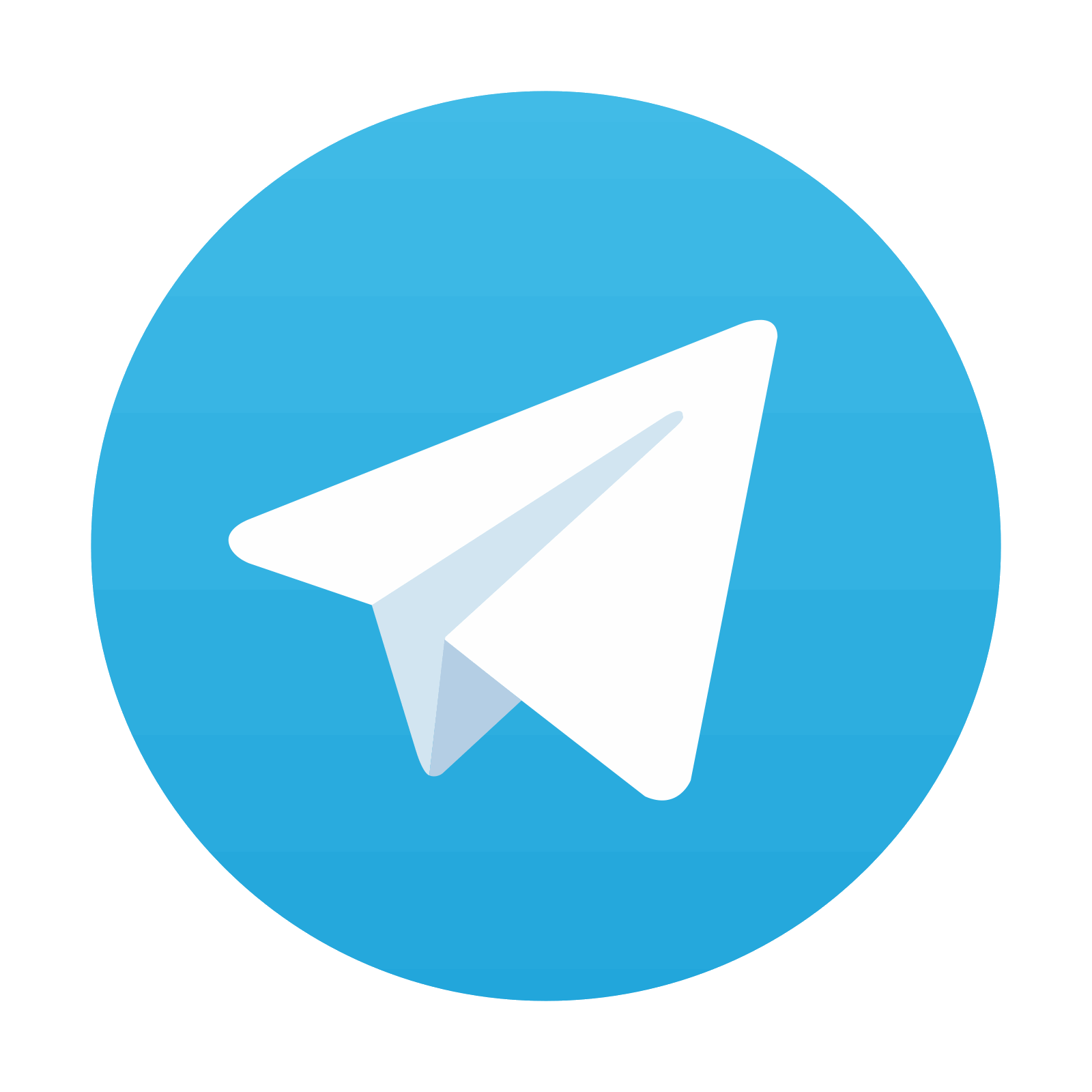
Stay updated, free articles. Join our Telegram channel
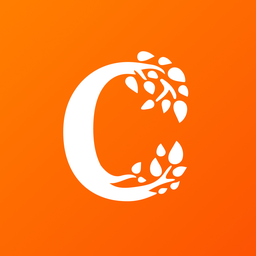
Full access? Get Clinical Tree
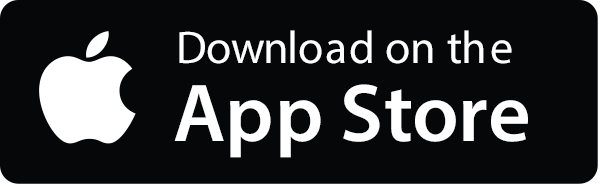
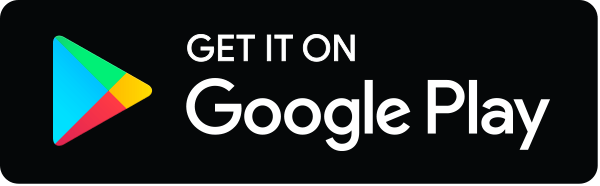