© Springer Science+Business Media New York 2016
Diana Vaamonde, Stefan S du Plessis and Ashok Agarwal (eds.)Exercise and Human Reproduction10.1007/978-1-4939-3402-7_66. General Adaptations to Exercise: Acute Versus Chronic and Strength Versus Endurance Training
(1)
Division of Exercise Science and Sports Medicine Department of Human Biology, University of Cape Town, 1 Boundary Rd, Newlands, 7725 Cape Town, Western Cape, South Africa
Keywords
Resistance trainingResistance to fatigueDetrainingHomeostasisExercise-induced adaptationsNervous systemIntroduction
A stressor is defined as anything that causes stress or elicits the stress response. A common feature of all stressors is that there is an acute phase, during which homeostatic adjustments occur; a more chronic phase, during which the stressor can be accommodated by the adaptations ; and finally a phase of exhaustion, during which maladaptations occur [1]. According to this definition, exercise can be defined as a stressor, along with other examples such as food deprivation, exposure to hypo- or hyperthermia, or psychological and social challenges. The stress of exercise behaves in a biological way similar to other stressors. For example, at the start of exercise, there are acute physiological, metabolic, and neuromuscular changes that persist for the duration of the bout of exercise. These changes are in proportion to the increase in metabolic rate. During rest, oxygen consumption, which is a measure of metabolic rate, hovers around 3 ml oxygen/kg/min, and coinciding with exhaustive exercise, oxygen consumption may increase to around 50 ml oxygen/kg/min in a moderately active person and to around 70 ml oxygen/kg/min in a highly trained endurance athlete. Almost immediately after exercise ceases, oxygen consumption decreases returning to baseline levels a few hours later. These changes during exercise are transient. For example, the metabolic state a few hours after moderate exercise is indistinguishable from the metabolic state before the start of the exercise.
However, if the bout of exercise is repeated regularly, after a few weeks changes occur, which persist for longer than the transient acute changes. These more persistent changes, known as training-induced adaptations, are associated with an improvement in exercise performance. For example, a trained person has an increased capacity to resist fatigue, has stronger muscles, can generate more muscle power, or has more refined motor coordination or skill for a specific task. The exact nature of the adaptations depends on the type of exercise training. Consider, for example, a typical endurance training session where the active muscles are recruited several thousand times to contract at a relatively low intensity. Indeed the leg muscles of a jogger, at the end of a 10-km run, would have had to sustain over 6000 contractions. In contrast, a person undergoing resistance training in a gym may only contract a muscle 30 times at each training session; the difference being that each contraction is close to a maximal effort. It is therefore not surprising that the training-induced adaptations are different, considering the different types of training stimuli.
Following each training session, recovery has to occur before the next training session. The time course of recovery after exercise varies depending on the marker of recovery. For example, elevated heart rate, blood lactate concentrations, and breathing rate return to baseline levels within minutes after exercise, whereas metabolic rate may take hours. If the bout of exercise caused muscle damage, the changes may take weeks [2] or months [3] to revert to the pre-exercise values. Inadequate recovery between training sessions results in maladaptations with the accompanying symptoms of fatigue and impaired muscle function [4]. This is commonly known as overtraining and is something that has to be carefully managed, particularly with competitive athletes who are continually striving to improve performance by training hard with insufficient recovery between training sessions [5].
The principles of biology, particularly the principle of “dose and response” can be applied to exercise; the dose of exercise being the stimulus of a training session, and the response being the outcome from that training session. Coaches have learnt to apply this principle to training. Aligned to this principle is the concept of overload, which suggests that the biological stress has to be gradually increased for adaptations to occur. In practical terms, this translates to the weight lifted getting heavier, the distance run getting longer, or the intensity of the exercise increasing in a gradual, systematic way. This principle forms the basis of all training programs and assists athletes in reaching peak performances [6]. The next section will consider the biological signal in more detail.
Biological Stimulus
At rest, the physiological, metabolic, and endocrine systems in the body are in a state of equilibrium. This equilibrium is disturbed as soon as the muscles start contracting. This disturbance of homeostasis evokes several responses, all designed to meet the demands of the increased metabolic rate or the need to produce muscle power. Examples of these transient changes are [7]:
-
Altered blood flow to the active muscles
-
Increased heart rate
-
Increased breathing rate
-
Increased oxygen consumption
-
Increased rate of sweating
-
Increased body temperature
-
Secretion of stress hormones such as adrenocorticotropic hormone (ACTH), cortisol, and catecholamines
-
Increased glycolytic flux
-
Altered recruitment of muscles
In short, these responses or homeostatic adjustments maintain the constancy of the body’s internal milieu during exercise. The magnitude and type of responses to exercise are dependent on the interaction of several factors such as the type of muscle action, duration of activity, and whether the person has been exposed to the activity. The ambient conditions, such as temperature, altitude, and wind speed, also have an impact on the homeostatic response. Finally, there are significant intraindividual differences in responses to the same exercise stimulus [8]. This explains why some people seem to adapt at a much faster rate than others, after exposure to the same training stimulus. The reasons for this can be ascribed to pre-training phenotype and pre-training autonomic function. There is also evidence that the training response can be modulated by the timing and composition of the nutritional intake [8].
The exercise-associated signal that induces training-induced adaptations varies depending on the type of muscle contraction and the duration, frequency, and intensity of the bout of exercise. This mechanical signal is converted into primary and secondary messages for adaptation. These messages activate the pathways involved in protein synthesis or degradation, resulting in the adaptations that are associated with changes in performance. Also, the recovery period between exercise sessions can affect the signal. For example, the training-induced expression of messenger RNA (mRNA) of several oxidative enzymes are upregulated at 24 h after the bout of exercise [9].
A consequence of endurance training is that the muscle becomes more resistant to fatigue [10], whereas following resistance training , the muscles become stronger, more powerful, and in some cases bigger [11]. At the cellular level, the primary stimulus for exercise-induced adaptations is a combination of the load on the muscle, metabolic stress , and calcium flux [12]. The load of the muscle is dependent on the tension or strain and type of muscle action. For example, the muscle can shorten or lengthen under tension [13]. A muscle that lengthens under tension has unique activation patterns [14], which impact the mechanical signal. Increasing the load causes muscle hypertrophy through the activation of the pathways of protein synthesis and a suppression of the protein degradation pathways. This results in an increase in the amount of the myofibrillar contractile proteins (actin and myosin), with an increase in muscle cross-sectional area [11].
The metabolic stress stimulus varies depending on the intensity and type of exercise [15]. Metabolic stress is associated with a high rate of adenosine triphosphate (ATP) utilization and is influenced by the substrate availability in the muscle cell. The biochemical signals that activate adaptive cellular pathways include an increased ratio of adenosine monophosphate (AMP) to ATP (ATP), increased levels of reactive species of oxygen and nitrogen, depleted levels of muscle glycogen, and decreased oxygen tension [15].
The calcium flux refers to the rate at which calcium is released from the sarcoplasmic reticulum, binds to troponin C, and initiates muscle contraction [16]. During a single-muscle contraction, there is a transient increase in sarcoplasmic calcium concentration. However, as the rate of muscle contractions increases, the sarcoplasmic calcium concentrations are maintained at a higher level. The latter scenario is associated with a signal that results in mitochondrial biogenesis and an increase in the glucose transporter (GLUT 4) [17]. The increase in mitochondrial content and oxidative enzyme activity results in a reduced rate of glycogen utilization during exercise and an enhanced capacity to oxidize fat during submaximal exercise. This results in an increased capacity to resist to fatigue and is associated with enhanced endurance performance.
In summary, these three stimuli (load on the muscle, metabolic stress, and calcium flux) vary depending on the circumstances of the exercise. During endurance training, the load is low, the metabolic stress is high, and the calcium flux consists of short intermittent bursts for long periods. Under these conditions, there is an increase in mitochondrial mass and oxidative enzyme activity. During resistance training (i.e., few contractions in a training session, but these contractions are close to maximal power output), the load is high, the metabolic stress is moderate, and the calcium flux is high. This stimulus results in muscle fiber hypertrophy and neural changes. If the person exposed to resistance is untrained, almost all the changes in strength can be attributed to neural changes in the early phases of training . However, after 4–6 weeks of training, the first signs of hypertrophy become evident. This will be discussed in more detail in the section on resistance training.
Finally, there is evidence to suggest that a slightly elevated intramuscular temperature is an important part of the signal for inducing training adaptations . This was concluded after an experiment showed that training-induced adaptations were blunted if the muscles were cooled immediately after each training session [18].
Maladaptations
For competitors striving to improve performance, there is a fine line between doing insufficient training or too much training, with both resulting in underperformance. Insufficient training fails to induce appropriate adaptations necessary for peak performances. In contrast, too much training results in maladaptations or the failure to adapt, causing symptoms of fatigue and poor performance. This has been well researched and it is known that training-induced fatigue occurs on a continuum starting with “functional overreaching,” which is overcome with a few days of rest [5]. Indeed some coaches will even induce this state intentionally to capitalize on the rebound and possible super compensatory effect as the athlete recovers. The next phase is “nonfunctional overreaching,” which requires a longer rest period. This is regarded as a negative phase, which coaches try and avoid. If the training load persists, without adequate recovery, the athlete enters the overtraining phase [19]. A consistent symptom of overtraining is impaired performance. However, beyond this, the symptoms of overtraining are varied and may involve dysfunction in the neuromuscular, endocrine , metabolic, and immune systems. A common characteristic is the inability to accommodate the same training load that was possible before the development of the symptoms [20]. Symptoms of overtraining can be avoided if the athlete adopts a more systematic approach to training, ensuring the correct balance between training load and rest and recovery [21]. To facilitate this process, it is important to quantify both the training load and the fatigue arising from each session, so that the “dose/response” can be carefully manipulated [22]. This approach will increase the chances of the athlete peaking at the correct time coinciding with important competition. Researchers have attempted to identify a specific marker of overtraining—at present such a marker does not exist, possibly because of the varied and inconsistent responses [5].
Endurance Training
The main consequence of training-induced adaptations following endurance training is that resistance to fatigue increases . This means that after training more work can be done in the same time, compared to before training, or at the same submaximal intensity, the time to fatigue increases after training. Although the adaptations after endurance training are loosely referred to as aerobic adaptations, they have their origins in the skeletal muscle, in the cardiovascular system and in metabolism. Aerobic capacity is represented by the maximal oxygen uptake (VO2max). As mentioned previously, the VO2max of elite endurance athletes (males) is usually above 70 ml oxygen/kg/min, whereas it is about 10 % lower in elite females. Although VO2max increases following endurance training, the changes are variable, possibly as a result of the athlete’s genotype [15]. There are two schools of thought explaining the increase in VO2max after training. The first view is that VO2max increases as a result of the central adaptations that result in more oxygen being delivered to the muscles. According to this view, the changes in performance are a consequence of the increased oxygen delivery [23]. A second view is that the properties of the muscle change after training, enabling the person to achieve a higher workload in a VO2max test. It follows that VO2max increases as a consequence of the increased workload [24]. The latter interpretation seems to be the more popular and is supported by basic principles of exercise physiology [25].
If one considers a situation where an inactive person starts training systematically, there are a number of changes that take place. One of the first measureable changes is an increase in plasma volume [26]. This adaptation can also explain the increase in cardiac output and stroke volume and the decrease in heart rate, which occurs during submaximal exercise after training [27]. These changes are lost as soon as the regular training stops. Next, the capillaries in the active muscles proliferate. The capillary-to-fiber ratio increases by up to 20 % in a matter of weeks [28]. Another early adaptation evident after about 4 weeks of training is the increase in the mitochondrial mass with a concomitant increase in oxidative capacity [29].
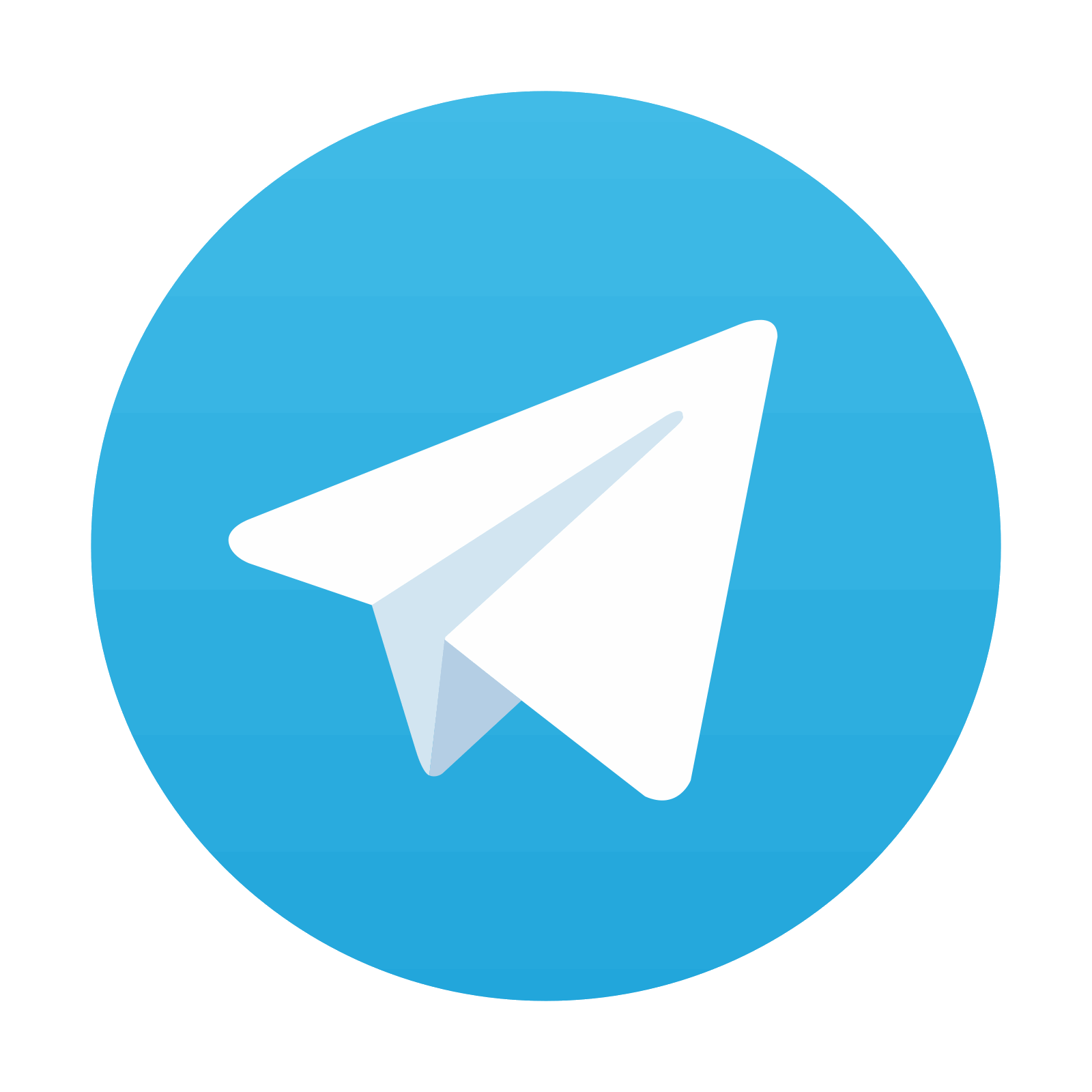
Stay updated, free articles. Join our Telegram channel
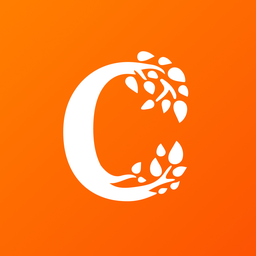
Full access? Get Clinical Tree
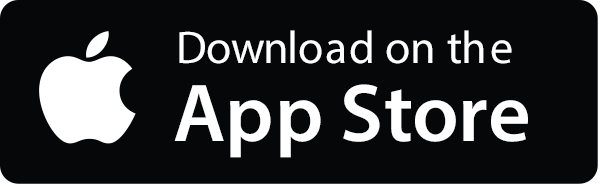
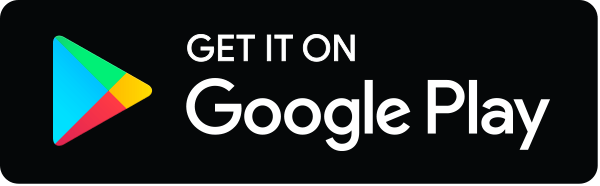