Fig. 4.1
Fibroblast growth factor receptor 1 (FGFR1) signal transduction. The binding of FGF-1, -2, or -4 at the interface of the IgII and IgIII domains, together with the binding of heparin or heparan sulfate to the acid box, induces dimerization and subsequent activation of FGFR1. Autophosphorylation of seven tyrosine residues in the intracellular domain of the receptor triggers the activation of three major signaling cascades. The mitogen-activated protein kinase (MAPK) pathway is activated through the binding of the FGFR substrate (FRS)-2 adaptor protein and sequential activation of Ras-Raf-MEK1/2 kinases. The Akt pathway is induced via FRS2-mediated recruitment of the adaptor protein Gab1 and activation of PI3K. The protein kinase C (PKC) pathway is activated by direct binding of phospholipase C (PLC)-γ to Tyr766 of FGFR1. Active PLC-γ hydrolyses phosphatidylinositol-4,5-diphosphate (PIP2) to diacylglycerol (DAG) and inositol-1,4,5-triphosphate (IP3); DAG subsequently induces activation of PKC, and IP3 triggers the release of Ca2+ from intracellular stores
4.3 Alternative Splicing Yields Structurally and Functionally Diverse FGFRs
The complexity of the FGF/FGFR signaling network is additionally enhanced by alternative splicing, which results in the expression of multiple isoforms of a single FGFR family member. There is considerable structural diversity between the different FGFR splicing isoforms; for example, soluble, secreted FGFRs, FGFRs lacking the kinase domain, or receptors containing two or three Ig-like domains resulting in altered ligand binding specificity are produced [20, 21]. One of the most carefully studied splicing events in the FGFR protein family is the splicing of the messenger RNA (mRNA) coding for the C-terminal part of the D3 (IgIII) domain. This domain can be encoded in two different ways: isoform IgIIIb is encoded by exons 7/8, and isoform IgIIIc is encoded by exons 7/9. The alternative splicing of FGFR1, FGFR2, and FGFR3 produces both IgIIIb and IgIIIc variants, while FGFR4 splicing yields only the IgIIIc isoform. These isoforms confer different ligand-binding specificity, e.g. FGFR3IIIb binds to FGF-1 and -9, and the FGFR3IIIc isoform binds to FGF-1, -2, -4, -8, -9, -17, -18, and -23 [22]. Moreover, different FGFR isoforms display a cell-type-specific expression pattern, e.g. IgIIIb isoforms are predominantly expressed in epithelial tissues, and IgIIIc isoforms in mesenchymal tissues [23]. The most prominently expressed FGFR isoform in cultured human endothelial cells (HUVECs) is FGFR1IIIc, followed by FGFR3IIIc and FGFR2IIIc [15]. Moreover, the expression of FGFR1IIIc and FGFR2IIIc isoforms were shown in the human aorta [15].
4.4 Three Major Pathways are Involved in Canonical FGF/FGFR Signal Transduction
The FGF/FGFR signaling network is important in numerous cell types and has been studied more extensively in cell types other than endothelium. Typically, the signal transduction downstream of the FGFR involves three signaling mechanisms: mitogen-activated protein kinases (MAPKs), PI3K/Akt, and phospholipase C (PLC) [24]. In endothelial cells, signaling via the MAPK pathway and PI3K/Akt is well established, while the significance of FGFR-induced PLC-γ activation is still controversial [25]. Since the activation process is studied in greatest detail for FGFR1, and FGFR1 is the predominant FGFR isoform in endothelial cells, we will focus here on the description of activation and signaling elicited by FGFR1 (Fig. 4.1).
At the basal auto-inhibited state of FGFR1, a proline residue in the C-terminal part of the kinase activation loop prevents binding of the kinase substrate. Interestingly, ATP can bind to the nucleotide-binding site under these conditions, occuring even without ligand stimulation [26]. Binding of FGF-1, -2, or -4 to the interface of the IgII and IgIII domains, accompanied by binding of heparin to the acid box in the IgII domain, induces conformational changes in the FGFR1 structure. Heparin or heparan sulfate are required to stabilize the complex between the FGF ligand and the FGFR [27]. Subsequent dimerization of the FGFR results in autophosphorylation of seven cytoplasmic tyrosine residues [27, 28]. In addition, adaptor proteins of the FGFR substrate (FRS)-2 family—FRS2α and FRS2β—that are associated with the juxtamembrane domain of FGFR1 already in its inactivated state, become tyrosine-phosphorylated by the activated receptor [29]. The phosphorylated tyrosine residues now serve as docking sites for the recruitment of adaptor proteins and enzymes containing either a phosphotyrosine-binding domain (PTB) or an Src homology 2 (SH2) domain.
As mentioned above, FRS2α and FRS2β bind to the juxtamembrane domain of FGFR1 via their PTB domains, independently of the receptor activation status [30]. Upon activation of the FGFR1, FRS2α recruits other adaptor proteins, namely Shp2 and Grb2. Grb2 forms a complex with the guanidine nucleotide exchange factor (GEF) Sos, which in turn activates the small GTPase Ras. This event induces a whole cascade of protein kinases, finally leading to phosphorylation and activation of the MAPK ERK1/2, p38, and JNK. Among those, Erk1/2 kinases phosphorylate a wide range of cytoplasmic proteins (such as cytoskeleton-associated proteins) and transcription factors (e.g. c-Fos, Ets, Elk-1, etc.) and thereby regulate processes such as cell proliferation, differentiation and migration [31].
Activation of the PI3K/Akt pathway downstream of FGFR1 also depends on FRS2. In this pathway, upon FGFR1 activation, FRS2 recruits the adaptor protein Gab1, which leads to activation of PI3K and, subsequently, Akt. Active Akt kinase induces prosurvival signaling pathways, reflecting once more the diversity of cellular signaling events initiated by activation of FGFR1 [24].
Finally, PLC-γ binds to the phosphorylated tyrosine 766 in the C-terminal tail of FGFR1 via its SH2 domain [28]. In the next step, activated PLC-γ hydrolyses phosphatidylinositol-4,5-diphosphate (PIP2) to diacylglycerol (DAG) and inositol-1,4,5-triphosphate (IP3). Finally, DAG induces activation of the protein kinase C (PKC) and IP3 triggers the release of Ca2+ from intracellular stores, resulting in activation of Ca2+-dependent signaling events [24].
Beyond this canonical FGF signal transduction via the FGFRs, the exogenous FGF-1 and FGF-2 molecules have been shown to be able to reach the cell cytosol and nucleus; therefore it has been proposed that they have a dual role in signal transduction, partly independent of the FGFRs [32–34]. This has particularly been shown in the context of cell growth regulation and rRNA synthesis [35–37]; however, the molecular details of this signaling process and its importance for endothelial cell biology are not completely understood.
4.5 Negative Regulation of FGFR1 Signaling
In order to keep the FGFR1 signaling under tight control, a mechanism of negative regulation of signal transduction is required. This is achieved via recruitment of proteins such as Cbl, Sprouty (Spry), Sef, and MAPK phosphatases.
Upon activation of the FGFR1, the ubiquitin ligase Cbl is recruited via FRS2 and Grb2 adaptor proteins. Cbl subsequently ubiquitinates the FGFR1 as well as FRS2, leading to attenuation of FGFR1 signaling [38], possibly by regulating the trafficking of FGFR1 to the lysosome [39]. However, the process of endocytosis and sorting of activated FGFRs is not thoroughly investigated in endothelial cells. In epithelial cells, the activated FGFR1 is co-internalized with E-cadherin, and co-localization in EEA1- and Rab5-positive endosomes shows that both proteins share the initial endocytosis and sorting mechanism in this cell type [40]. Furthermore, in vivo studies in Xenopus indicated that extended-synaptotagmin-2 mediates the endocytosis of the FGFR1, most probably through clathrin-dependent endocytosis [41]. Finally, a series of studies by Wiedlocha and Sorensen have demonstrated that FGFR endocytosis can occur through both clathrin- and caveolin-dependent pathways [34].
An additional level of negative regulation of the activated MAPK pathway downstream of the FGFR is achieved through the action of the four members of the Spry protein family [42]. Spry proteins form homo- or hetero-oligomers, which can inhibit the MAPK pathway via interaction with the FRS2-Grb2-Sos complex or directly via interaction with Raf kinase [43]. Overexpression of Spry-1 or Spry-2 in endothelial cells has been shown to inhibit FGF-2-dependent MAPK activation [44].
The transmembrane protein Sef (similar expression to FGF) is another negative regulator of FGFR signaling. Sef is a direct inhibitor of tyrosine phosphorylation of the FGFR1, as well as several kinases in the MAPK pathway [45, 46].
Finally, MAPK phosphatases, also known as dual-specificity phosphatases (DUSP), are important for the attenuation of FGFR1 signaling [47–49]. Recently, it has been shown that ERK-mediated phosphorylation of Ser777 in the C-terminal tail of FGFR1 itself leads to inhibition of FGFR1 signaling, proliferation, and migration, and constitutes a negative feedback mechanism in the control of FGF signaling [50].
4.6 FGF Co-Receptors and Extracellular Matrix Components Contribute to FGF-Dependent Signal Transduction
The FGF co-receptors Klotho (one of the three fates in Greek mythology), Cfr (cysteine-rich FGFR) and Flrt (fibronectin leucine-rich transmembrane protein), localized on the extracellular face of the plasma membrane, can support the formation of certain FGF/FGFR complexes and promote the FGFR signaling activity [51–54]. The endocrine FGFs (FGF-19, -21, and -23), which have a low affinity to heparan sulfate, rely on Klotho as essential tissue-specific co-receptors [53]. Furthermore, proteins involved in cell-adhesion processes, such as integrins, neural cell adhesion molecule (NCAM), and N-cadherin, are involved in the regulation of FGFR signal transduction [55].
As mentioned above, components of the ECM , such as heparan sulfate proteoglycans (HSPGs), also perform an important regulatory function in FGFR signaling. HSPGs regulate the distribution of FGF ligands in the extracellular environment, and thereby the accessibility of FGFs for activation of its receptor [56]. One of the HSPGs, syndecan-4, has been shown to play a role in the regulation of FGFR signaling in endothelial cells. In their study, Elfenbein and Simons have shown that syndecan-4 promotes the internalization of the FGFR1/FGF-2 complex in endothelial cells. The authors described a mechanism whereby syndecan-4 mediates macropinocytosis of the FGF-2/FGFR1 complex and modulates the kinetics of FGFR1-dependent MAPK activation [57, 58].
Moreover, a study addressing a potential FGFR-independent role of syndecans in FGF signaling has been performed. In this study, overexpression of full-size syndecan-4 in human endothelial cells enhanced FGF-2-dependent signal transduction. In contrast, overexpression of the extracellular domain of syndecan-4 linked to the glycosylphosphatidylinositol (GPI) anchor sequence from glypican-1 failed to induce FGF-2 signaling [59]. Since the intracellular domain of syndecan-4 binds to several phospholipids and proteins, e.g. PIP2 and PKCα, the authors argued that the overexpression effects were due to the intrinsic FGF-dependent signaling properties of the full-size syndecan-4 protein [59], independent of FGFR1 [57, 58]. Additional experiments in rat fat pad endothelial cells (RFPECs) by the same group demonstrated that only the expression of full-size syndecan-4 was able to enhance the FGF-2-dependent signaling response [60]. Furthermore, it has been proposed by the same group that syndecan-4 is involved in the activation of the small GTPase Rac1 in endothelial cells [61, 62]. However, additional studies in alternative experimental settings are required in order to confirm the role of syndecans as FGFR-independent FGF signal transducers.
In addition to the co-receptors and ECM components mentioned, the biological activity of FGFs might be regulated by binding to gangliosides, thrombospondin-1, the fibronectin fragment fibstatin, or FGF-binding protein, as well as to a number of serum components such as soluble FGFR1, fibrinogen, α2-macroglobulin (α2 M), pentraxin-3, and several cytokines [5].
4.7 Current Knowledge About FGFs and Their Receptors in Vascular Development is Limited
In comparison to other ligand receptor pairs, such as vascular endothelial growth factor/vascular endothelial growth factor receptor (VEGF/VEGFR), Eph/EphR, or Ang/Tie, the function of FGFs and their receptors in the development of the vascular system is poorly understood [63, 64]. Loss-of-function studies in mice have not been informative because FGF-1 and FGF-2 knockout mice (both single and double knockouts) do not show a strong phenotype, both in respect to the vascular system as well as development in general [65]. This might be explained by the existence of various FGFs and their functional redundancy [63]. However, it has been reported more recently that FGF-2 knockout mice show a decreased number of lymphatic vessels in the eye cornea [66] (see 4.15), indicating that more specific defects in the vascular system might be observed upon detailed analysis. In addition, complete FGFR1 and FGFR2 knockout mice are developmentally arrested before the onset of vascularization [67–69], making the analysis of the function of FGFR1 and FGFR2 during vascular development impossible .
The Ornitz Laboratory has recently generated tissue-specific FGFR1/FGFR2 double knockout mice with deletion of both genes in endothelial/hematopoietic cells. These mice are viable, indicating that deletion of FGFR1 and FGFR2 in these cell types does not result in lethal developmental defects. An initial analysis of vascular developmental in these mice suggests that endothelial FGFR1/2 signaling is not strictly required for vascular development or homeostasis, but is important for neovascularization in response to injury (Ornitz D, personal communication). These mice represent a highly valuable tool for the future detailed study of FGFR1/2 signaling in the various aspects of vascular development.
To date, evidence for the function of FGFs in angiogenesis stems from a variety of in vitro and in vivo models. These will be discussed in the following section, including information regarding the underlying signaling mechanisms, where available.
4.8 The Vascular System is Built Through Vasculogenesis and Angiogenesis
The vascular system forms as one of the first functional structures during embryonic development . It is built through two interlinked, but mechanistically distinct, processes, referred to as vasculogenesis and angiogenesis . Vasculogenesis describes an assembly process of precursor-derived endothelial cells into a primitive vascular plexus, while angiogenesis is defined as the formation of new blood vessels from a pre-existing network [70]. The mesoderm is the origin of endothelial cell and, in amniotes, the differentiation occurs in several distinct regions: (i) in the embryo proper, where endothelial precursors are first detected within paraxial and lateral plate mesoderm around embryonic day (E)7.0 of mouse development; and (ii) in the extraembryonic mesoderm of the yolk sac, allantois, and placenta [71–73]. Cells from the extraembryonic splanchnopleuric mesoderm are induced to develop into hemangioblasts, which are common precursors of blood and endothelial cells and form the blood islands on the yolk sac around E7 [74]. In the following developmental process, the inner cells of the blood islands differentiate into hematopoietic stem cells surrounded by endothelial precursors, so-called angioblasts. From these angioblasts, the first blood vessels are formed de novo in the process of vasculogenesis . The intraembryonic vascular networks in amniotes, but also in fish and amphibians, arise from single angioblast progenitors situated in the mesoderm that surrounds a developing organ and these angioblasts, e.g. give rise to the dorsal aorta [74–76].
4.9 FGF is an Important Inducer of Angioblasts during Vasculogenesis
The endoderm provides the growth factors for the induction of the mesoderm; it was shown that one of the key players in the process of mesoderm induction is FGF-2 [77, 78], together with TGFβ [79] early studies perfomed in vivo on Xenopus embryos and in vitro on dissociated pre-gastrulation avian embryos identified FGFs as potent inducers of mesoderm and subsequent angioblast formation [74, 80]. Furthermore, the expression of dominant negative FGFR (Xenopus homolog of FGFR1, containing only the extracellular and transmembrane domains) in Xenopus disrupted mesoderm formation [81]. Cox and Poole employed vasculogenesis assays using quail/chick chimeras, where somite-derived mesodermal precursors were transplanted from a quail donor and gave rise to angioblasts in a chick recipient. In this experimental setting, the injection of FGF-2 -blocking antibodies, along with the quail somite, significantly reduced the number of angioblasts originating from the somite. By implantation of beads soaked with FGF-2 into quail/chick chimeras, the authors further demonstrated that FGF-2 not only regulates the angioblast induction from uncommitted mesoderm but is also required to establish an initial vascular pattern in the developing embryos [82]. The importance of FGF-2 for vasculogenesis was also confirmed in cultures of quail blastodisc-derived cells. These cells efficiently differentiated into blood vessels only in the presence of FGF-2 [83].
In contrast to the embryonic stem-cell-derived embryoid bodies from avian and Xenopus embryos, mouse embryoid bodies are less suitable for studying the involvement of single growth factors in angioblast induction because they have a tendency to spontaneously undergo vasculogenesis. However, the addition of a growth factor cocktail, including FGF-2, significantly increases the development of primitive vascular-like structures from mouse embryoid bodies [84]. The important function of FGF-2 in inducing vasculogenesis has recently been confirmed in a study using mouse embryonic stem cells or embryoid bodies. Treatment with α2 M induced both vasculogenesis as well as angiogenesis via induction of FGF-2 expression. Incubation with the FGFR1 inhibitor SU5402 prevented the α2 M-dependent activation of both vasculogenesis and angiogenesis [85]. Furthermore, FGF-2 in combination with leukemia inhibitory factor (LIF) has been shown to induce vasculogenesis in endothelial cells derived from mouse embryonic stem (ES) cells in vitro [86].
Interdependence of the FGF and VEGF signaling network in the regulation of vasculogenesis (and angiogenesis) was shown in experiments performed on explanted mouse embryonic hearts. In this setting, FGF-1, -2, -4, -8, -9, and -18 induced coronary vasculogenesis as well as angiogenesis. The provasculogenic/angiogenic effects of all tested FGFs in this assay were dependent on VEGF signaling and vice versa [87], indicating an extensive crosstalk and synergism between FGFs and members of the VEGF family, which we will continue to discuss below (4.16).
4.10 Developmental Angiogenesis is a Multistep Process Producing Stereotypical Vascular Patterns
Developmental angiogenesis is a highly stereotypical process, which leads to the establishment of organ-specific vascular patterns with reproducible anatomy [88]. The process of angiogenesis can be broken down in a series of events, namely destabilization of an existing vessel and degradation of the ECM, proliferation and directed migration of endothelial cells, the formation of new endothelial cell/cell contacts and vascular tubes followed by coverage with pericytes or vascular smooth muscle cells and vessel stabilization [89]. The functional unit in the process of establishing new vascular branches is the angiogenic sprout, which consists of several types of specialized endothelial cells. The leading position of the vascular sprout is taken by the so-called tip cell. Tip cells are highly polarized and form numerous cellular protrusions, referred to as filopodia [90]. Through these filopodia, the tip cells constantly sense the microenvironment for guidance cues in order to navigate the growing vessel. Tip cells also regulate capillary branching by detecting and connecting to neighboring sprouts. Sensing of the directional cues through filopodia, as well as translation into directed migration, strongly depend on coordination of the tip cell cytoskeleton with membrane dynamics and the activity of small GTPases is thought to be important for tip cell function [91–95]. Stalk cells follow the leading tip cell; they proliferate and thereby elongate the growing branch [88, 90, 91]. However, the assignment of these specialized functions is only transient, and endothelial cells dynamically shuffle their relative positioning in the angiogenic sprout, probably due to continuous competition for the tip cell function [96]. Finally, quiescent phalanx cells build the inner lining of the new vessel after its outline has been set [88, 90, 91].
4.11 The Role of FGF in Vessel Destabilization and Matrix Remodeling
During the first phase of angiogenesis, degradation of the ECM is an important step, and matrix metalloproteinases (MMPs), as well as the plasmin/plasminogen system, have been implicated in this process [5, 97, 98]. Urokinase plasminogen activator (uPA) converts the inactive proenzyme plasminogen into the active serine protease plasmin, which degrades fibrin and other ECM components [99]. FGF-1, FGF-2, and FGF-4 induce uPA expression in endothelial cells and, in addition, enhance the cell surface expression of the uPA receptor, which might mediate localization of the proteolytic activity to the site of active migration [100]. In line with these findings, FGF-2-induced vessel formation was decreased on the cornea of uPA and plasminogen knockout mice [101], suggesting an important role of the plasmin/plasminogen system in mediating the proangiogenic FGF signal.
The expression of several MMPs is also positively regulated by FGFs [5, 98]. Importantly, FGF-2 stimulation leads to increased shedding of pro-MMP-2 and -9 as well as MT1-MMP-containing vesicles from endothelial cells, thereby enhancing proteolytic activity in the pericellular compartment [102]. Notably, MT1-MMP is highly expressed in tip cells [103]. These MMPs degrade capillary basement membrane components such as type IV collagen or HSPGs, but also serve to reveal cryptic proangiogenic binding sites, e.g. for integrins [98]. In addition, MMPs, such as MMP-3 and -13, as well as plasmin have been shown to liberate HSPG-tethered FGF-2 from the matrix, demonstrating the intricate interplay between growth factors, proteinases, and the ECM [98, 101, 104].
4.12 FGFs in Angiogenic Sprout Formation and Function
As described above, the angiogenic sprouting process requires the complex action of a number of different specialized endothelial cells, which need to coordinate a variety of distinct cellular functions, such as matrix degradation, proliferation, migration, and regulation of cell/cell contacts. Therefore, it is important to analyse the role of proangiogenic factors, such as FGFs, in a system that allows for these complex cell/cell interactions in a setting most closely related to the in vivo situation.
FGFs promote sprouting angiogenesis from embryoid bodies [105]. FGF-2 has been shown to induce angiogenesis from pre-existing vessels in the chorioallantoic membrane (CAM) and the yolk sac membrane of the developing chick [106, 107], and FGF-2 administration on the quail CAM induces vessel growth from small vessels in the arterial tree [108]. Interestingly, FGF-2 application induced a strong angiogenic response at E7, when FGFR expression was high, but FGF-2 response was much less pronounced at later embryonic stages, when FGFR expression was decreased [108]. Likewise, angiogenesis is induced in endothelial corneal cells after implantation of FGF-2-containing hydrogel into the rabbit mid-stroma corneal pocket [109]. It has also been demonstrated that endogenous FGF-2 induces vessel outgrowth from heart explants [110]. Moreover, the administration of FGF-2 into the perivitelline space of developing zebrafish embryos induced dose-dependent vascular sprouting from adjacent subintestinal vein vessels [111].
In addition to these model systems, a number of mouse models have been developed that allow us to study the role of the FGF/FGFR system in vascular development. General overexpression of FGF-2 in transgenic mice leads to a so-called ‘latent angiogenic phenotype’ characterized by a predisposition to angiogenic reactions and amplified angiogenesis demonstrated in the matrigel plug assay [112]. In line with these experiments, overexpression of FGF-2 in the retina of transgenic mice has been reported to cause a similar proangiogenic phenotype in the context of cell injury [113]. In accordance with this proangiogenic role of FGF-2, the adenovirus-mediated delivery of FGF-2-antisense RNA to endothelial cells of mouse embryos cultured ex utero disrupts vascular development. Major phenotypes observed after FGF-2 knockdown at E7.5 included abnormal development of the yolk sac vasculature and growth cessation, which were reversed by application of FGF-2 complementary DNA (cDNA) [114]. The same group has circumvented the early lethality of FGFR1 knockout mice, and used adenoviral delivery of a dominant negative FGFR1 mutant to endothelial cells of E9 mouse embryos cultured ex utero. The disruption of FGFR1 function caused defects in developmental angiogenesis, including incomplete branching of the yolk sac vasculature and defects in intersomitic vessels and brain vascularization [115], demonstrating an important role of FGFR1 in these developmental processes. More recently, a role for FGFR2 has been suggested (in the mouse heart) since endothelial-cell-targeted overexpression of constitutively active FGFR2 induces increased migration and tube formation in isolated endothelial cells, and conveys cardioprotection and enhanced angiogenesis after myocardial infarction [116].
4.13 Function of FGFs in Proliferation and Migration of Endothelial Cells
The complex nature of the angiogenic process entails that the role of FGFs in its discrete steps is difficult to analyse in the physiologically relevant in vivo situation. Therefore, in vitro cell culture systems for endothelial cells are employed, which allow single aspects of cell behavior to be specifically addressed. Although there is a certain caveat concerning the significance of the results obtained in these in vitro settings, they have allowed valuable insight into the involvement of FGFs in the key processes of angiogenesis.
In 1984, FGF-2 purified from chondrosarcoma ECM has been shown to stimulate the proliferation of capillary endothelial cells in vitro, and the proproliferative action of FGF on endothelial cells has subsequently been confirmed by a large number of laboratories [2, 3, 16]. FGF-2-induced proliferation of immortalized capillary endothelial cells is accompanied by tyrosine phosphorylation of the adaptor protein FRS2, which in turn interacts with Grb2, leading to strong and sustained activation of the MAPK ERK2. Accordingly, the addition of the MEK inhibitor PD98059 suppressed MAPK activity, which inhibited the proliferative response to FGF-2 [117]. Moreover, proliferation of choriocapillary endothelial cells has been shown to be stimulated by FGF-2 in vitro and required both ERK1/2 and PI3Kinase/Akt pathways, demonstrating the importance of these signaling pathways for FGF-2-induced proliferation in endothelial cells [118]. In contrast, FGF-2-induced activation of the p38 pathway in endothelial cells is involved in the negative regulation of cell survival, proliferation, and differentiation [119]. Moreover, FGF-2-dependent Akt signaling has been shown to be an important survival signal in endothelial cells [120].
The coordinated movement of tip-cell-directed angiogenic sprouts is crucial for the establishment of the vascular branching pattern; however, the role of FGFRs in the specification of endothelial tip versus stalk cells and/or the guidance of vascular sprouts is not entirely clear. The function of FGF/FGFRs in directed migration and branching is most extensively studied in the Drosophila airway system, which is highly reminiscent of tip-cell-guided developmental angiogenesis. Here, FGFs determine the specification of the epithelial tip cell, which directly responds to the FGF ligand and leads airway branch outgrowth [91]. Accordingly, in a mixed endothelial cell population, in which FGFR1 was silenced in half of the cells, FGF-2-responsive cells lead migration, whereas nonresponsive cells adopted a subsidiary trailing position in the context of sheet migration [121]. Interestingly, an FGFR1 mutant lacking the C-terminally located 63 amino acids of the cytoplasmic domain failed to mediate chemotaxis in a modified Boyden chamber assay, but efficiently mediated MAPK activation and FRS2 phosphorylation. In addition, the migration defect was independent of PLC-γ1 or phospholipase A2 activation, but sensitive to PI3K inhibition, suggesting a possible involvement of PI3K/Akt in mediating endothelial cell migration in this experimental setting [122].
The role of the FGF/FGFR system in endothelial cell migration is further highlighted by the fact that endothelial cells isolated from FGF-2 knockout mice have a defect in migration in response to mechanical damage in the so-called scratch assay in vitro. The migration defect is accompanied by reduced MAPK activation, and can be rescued by the addition of exogenous FGF-2 [123]. In addition, FGF-2 (alongside other FGFs) stimulates migration of endothelial cells from heart explants in the context of coronary angiogenesis. Importantly, coronary tubulogenesis of embryonic epicardium is responsive to many FGF family members and requires both FGF and VEGF-A [124]. Finally, expression of the dominant negative FGFR1 mutant (used by Lee et al. [115] in embryos ex utero as described above) in HUVECs leads to an impairment of MAPK signaling, reduction of endothelial cell number, induction of apoptosis and inhibition of migration in the modified Boyden chamber assay, confirming the role of FGF-2/FGFR1-initiated MAPK activation in these endothelial key properties [115].
In accordance with the well established role of small GTPases in the control of cell movement, FGF-2-induced angiogenesis in the in vivo matrigel plug assay depends on the presence of the small GTPase Rac [125]. Our laboratory has recently shown that FGF-2-induced activation of Rac1 in primary mouse lung endothelial cells depends on the presence of the F-BAR protein NOSTRIN. NOSTRIN serves as a membrane-bound multivalent adaptor to assemble a signaling complex containing FGFR1, Rac1, and its activating exchange factor Sos-1. The loss of NOSTRIN in NOSTRIN knockout mice, or after morpholino-mediated knockdown in zebrafish embryos, leads to reduced endothelial cell proliferation and directed migration, with a pronounced defect in tip cell filopodia formation, suggesting an important role for the FGF-2/FGFR1/Rac1 axis in regulating angiogenic sprouts in vivo [92]. Moreover, PI3K has been shown to regulate the activity of the small GTPases Cdc42 and Rac via activation of the GEF proteins [126], which are thought to be vital for mediating the directed migration of endothelial cells [91].
4.14 Function of FGFs in Capillary Branching and Vessel Maturation
To date, there has been no general scheme established describing the function of FGF signaling in branching morphogenesis; however, there is ample evidence for an important role of FGF during this process [127]. Upon overexpression of FGF-1 in the myocardium of transgenic mice, coronary artery density and branching are increased [128]. Likewise, overexpression of FGF-2 in transgenic mice leads to increased vessel density and vessel arborescence in the heart [129], illustrating the properties of these FGFs to positively regulate vascular branching. This has been confirmed by studies indicating that FGF-2 is a key determinant for vascular branching in luteal angiogenesis [130]. FGF-dependent signaling involves FGFR1, since a dominant negative version of FGFR1 expressed in retinal pigmented epithelial cells leads to a poorly branched vascular bed in the choroid and an avascular neonatal retina [131]. In accordance with these findings, embryoid bodies derived from FGFR1 knockout mice are characterized by abundant, but morphologically distinct, elongated vessels [132–134], pointing to the fact that FGFs are important for the establishment of vascular network morphology.
After completion of the early, invasive phase of angiogenesis, the late phase of angiogenesis serves the maturation of the newly formed vessel, and requires the formation of tight cell/cell contacts, deposition of ECM, and recruitment of pericytes or smooth muscle cells. FGFs have been suggested to contribute to these processes, e.g. through the regulation of integrin and cadherin expression and ECM deposition [5, 89]. FGFs play an important role in the formation and, more explicitly, the maintenance of cell/cell contacts. Inhibition of FGF signaling through the introduction of soluble FGF traps or dominant negative FGFR mutants in vitro and in vivo leads to dissociation of adherence and tight junctions followed by loss of endothelial barrier function and vascular integrity in adult mice [135]. The loss of FGF signaling disrupts the VE-cadherin-catenin complex at adherence junctions, and FGF-dependent regulation of expression and stability of the protein tyrosine phosphatase Shp2 have been implicated in this process [136]. However, the role of FGFs in maturation of newly formed vessels during developmental angiogenesis has not been investigated in detail.
4.15 FGFs and Lymphangiogenesis
While angiogenesis is a term used to describe development of the novel blood vessels from the pre-existing vasculature, lymphangiogenesis is a process of formation of novel lymphatic vessels. FGF-2, together with VEGF-C and VEGF-D is a potent lymphangiogenic factor. Analysis of FGF-2 knockout mice showed a decreased number of lymphatic vessels in the eye cornea compared with wild-type mice [66]. Application of FGF-2 was shown to induce lymphangiogenesis in mouse cornea in a dose-dependent manner. At lower doses, FGF-2 promoted formation of lymph vessels, whereas the endothelial angiogenic response was neglectable. The mechanism of FGF-2-induced lymphangiogenesis in this assay was dependent on the increase in expression of VEGF-C and VEGF-D [137, 138]. Moreover, VEGFR3 was shown to be important for FGF-2-dependent lymphangiogenesis in the mouse cornea [139]. A study by Matsuo et al. applying chemical inhibitors, suggested that FGF-2-induced lymphangiogenesis in temperature-sensitive rat endothelial lymphatic cells (TR-LE cells) was mediated via the Akt/mTOR/p70S6 kinase pathway [140]. More detailed experiments on FGF-2-mediated signaling in lymphangiogenesis were performed in primary lymphatic endothelial cells (LECs) isolated from embryonic mouse skin. These data show that FGFR1 is the primary receptor for FGF-2 in LECs, and that the FGF-2/FGFR1 signaling axis mediates proliferation and migration of LECs, while VEGF signaling is important for LEC sprouting and elongation [141]. In addition to FGFR1, additional FGFR isoforms might be important for FGF-2 signal transduction in LECs [142]. Several recent studies confirmed the crucial role of FGF-2/FGFR1 signaling in tumor lymphangiogenesis and proposed a potential treatment strategy for the inhibition of cancer metastasis [143, 144].
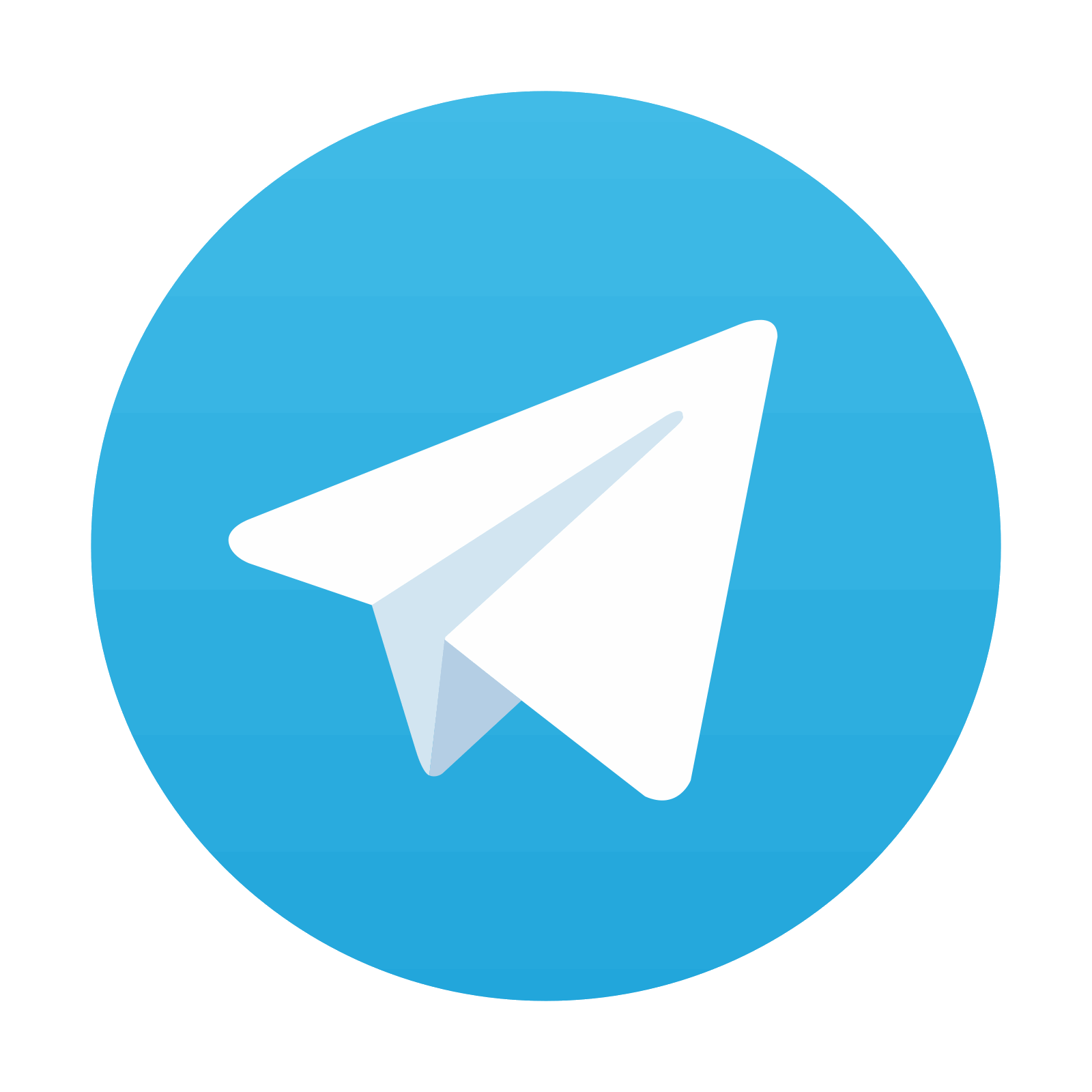
Stay updated, free articles. Join our Telegram channel
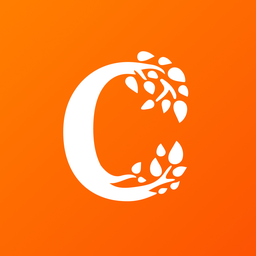
Full access? Get Clinical Tree
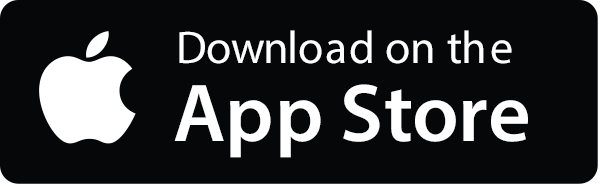
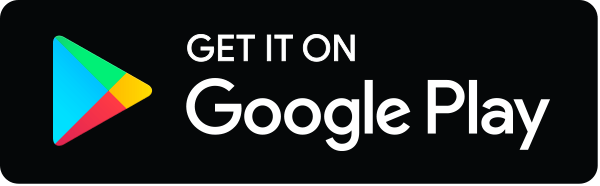