17 Thomas R. Everett1 and Christoph C. Lees2,3,4 1 The Leeds Teaching Hospitals NHS Trust, Leeds, UK 2 Imperial College London, London, UK 3 Queen Charlotte’s and Chelsea Hospital, London, UK 4 KU Leuven, Belgium Fetal growth restriction remains a major cause of perinatal mortality and morbidity, with over 30% of stillbirths having suboptimal growth as a contributory factor. The aetiology of growth restriction is multifactorial and there remains no robust means of predicting fetuses at high risk. This is, in part, due to the fact that the underlying causes are diverse. There also remains no single definition and it is becoming increasingly evident that fetal growth trajectory is as important (and in many case more important) than a single measurement. The balance between iatrogenic prematurity and consequent developmental problems and increased maternal interventions must be weighed against the increased risk of stillbirth. At preterm gestations, management protocols using fetal Doppler and computerized cardiotocography (CTG) improve outcomes, whilst at term gestations delivery does not increase poor outcomes; between 32 and 37 weeks’ gestation the evidence for optimal management remains to be established. In order to reduce stillbirth rates further, there remains a need to improve detection of growth restriction and further refine current management protocols. There is no single definition of a small or growth‐restricted fetus. Variably, some have considered the fetus with an abdominal circumference or estimated fetal weight (EFW) below the 10th centile as small for gestational age (SGA), whilst others have used the 5th or 3rd centile or required Doppler abnormalities to also be present. It is clear that not only a single measurement is important, but fetuses that have significant reductions in growth velocity are at increased risk even if the biometry is still within normal limits [1]. A recent multinational expert consensus statement defining placental fetal growth has set out definitions of early (<32 weeks) and late (>32 weeks’ gestation) growth restriction [2] (Table 17.1). Table 17.1 Definition of fetal growth restriction. Source: adapted from Gordijn et al. [2]. AC, abdominal circumference; CPR, cerebroplacental ratio; EDF, end‐diastolic flow; EFW, estimated fetal weight; PI, pulsatility index. The most evident consequence of growth restriction in a fetus is stillbirth. Suboptimal fetal growth is thought to be a significant contributor in 30–50% of antepartum stillbirths, of which placental dysfunction is likely the primary cause. SGA fetuses are at risk of premature delivery, both spontaneous and iatrogenic. Prematurity itself carries gestation‐dependent risks, particularly below 30 weeks’ gestation, and at this point SGA fetuses are at increased risk of these complications, estimated to be equivalent to being born about 2 weeks prematurely to actual birth gestation. Complications of prematurity include neonatal death, intraventricular haemorrhage, necrotizing enterocolitis and lung disease, which may be chronic. Low birthweight, particularly when associated with prematurity, has long‐term consequences for health and effects are seen particularly in neurodevelopmental outcomes and cardiovascular and metabolic changes. The Barker hypothesis was developed from the historical cohort related to the Dutch famine of 1944. It proposes that the in utero environment, particularly with low birthweight babies, causes ‘fetal programming’ to optimize fetal well‐being, but has long‐term deleterious effects on cardiovascular and metabolic well‐being. Whilst compelling, it should be noted that further factors including genetics and postnatal environment are also important in contributing to long‐term health outcomes. Although the phenomenon of shunting blood to the brain in a compromised fetus is sometimes termed ‘brain‐sparing’, it might best be considered as cerebral redistribution as abnormal brain development can be found in these children. Paediatric follow‐up of growth‐restricted fetuses shows poorer motor and cognitive function and lower IQ in these children. This is particularly the case when there has been fetal Doppler evidence of cerebral redistribution or evidence of significant placental dysfunction such as absent or reversed umbilical artery end‐diastolic flow (EDF) [1,3]. There is also evidence of reduced grey matter, reduced myelination, thinner cortex, reduced number of neurones and smaller head circumference in children born with growth restriction. However, other studies have not replicated these findings and the final determination on whether cerebral redistribution is independent of birthweight and gestation and related to abnormal neurodevelopment remains unclear [2,4]. Impaired angiogenesis results in reduced arteriole and capillary formation. As a consequence there is increased vascular resistance and hypertension. Endothelial dysfunction is evident with increased intimal thickness and vascular stiffness. Consequently, as well as hypertension, there is increased risk of earlier onset cardiovascular morbidity in adulthood [2,5]. Low birthweight is associated with an increase in adult type 2 diabetes and increased insulin resistance and dyslipidaemia has been shown in animal models of growth restriction. The regulation of fetal growth is complex. A fetus has genetically predetermined optimal growth, but the actual fetal growth is determined by the influence of maternal health, placental function and environmental factors. Embryonic growth in the first trimester is remarkably constant. As uteroplacental circulation becomes the major determinant of fetal growth, from the early second trimester discrepancies in fetal growth may become apparent. This is even more so the case in the third trimester. The function of the placenta is to facilitate oxygen and nutrient transfer to the fetus, whilst removing waste products. Early adaptation of the spiral arteries and trophoblast invasion is essential. This process is not fully understood, but involves complex interactions between genetic and epigenetic programming as well as an appropriate maternal immune response. Failure of this process may result in suboptimal fetal growth, though this is not invariable. Abnormal spiral artery transformation may be associated with completely normal outcomes and vice versa. Unlike children and adults, glucose is the main source of energy to the fetus and over 95% in the fetus is derived from maternal plasma glucose. Placental glucose transfer is primarily mediated by the glucose carrier GLUT‐1 via a mechanism of facilitated diffusion [6]. The transfer of cholesterol, fatty acids and amino acids across the placenta is also closely regulated. There is evidence of increased glucose and fatty acid transfer in cases of increased growth, as seen in gestational diabetes. Conversely, in placentas of growth‐restricted fetuses there is evidence of deranged amino acid transfer. Insulin‐like growth factor (IGF)‐2 plays an important role in the regulation of fetal growth and amino acid transport. Postnatally, IGF‐2 is produced by the liver in response to growth hormone (GH). In utero, however, IGF‐2 is primarily synthesized within the cytotrophoblast layer of the placenta. IGF‐2 binds the IGF receptor (IGF‐R), of which there are two types, IGF‐1R and IGR‐2R. Binding of IGF‐2 to IGF‐1R results in promotion of growth, whilst binding to IGF‐2R results in degradation of IGF‐2. Notably, whilst the IGF2 gene is paternally expressed, the IGF2R gene is maternally expressed, demonstrating the opposing parental effects of growth promotion and growth restriction [7]. Regulation of growth by IGF‐2 is highlighted by two conditions: Beckwith–Wiedemann syndrome (BWS), an overgrowth syndrome, and Russell–Silver syndrome (RSS), a syndrome of growth restriction. In BWS there is gain of methylation resulting in biallelic IGF2 gene expression and subsequent overgrowth. Conversely, in RSS the loss of methylation results in biallelic loss of IGF2 gene expression and subsequent reduced growth. In some cases of BWS and RSS, there may be biallelic gain or loss, due to the relevant part of the chromosome carrying these genes being inherited from the same parent; this is referred to as uniparental disomy. BWS and RSS are examples of genomic imprinting and epigenetics. Epigenetics is the presence of modifications to the genome that affect gene regulation and which are heritable but potentially reversible. DNA methylation is the commonest form of modification, although more complex histone modification may occur. Over 100 genes have been found to be affected by imprinting; many are involved in fetal and placental growth or thyroid, insulin and glycogen metabolism, with variation between those maternally and those paternally expressed. The recognition of epigenetic influences supports the parental conflict hypothesis: maternally expressed genes are involved in resource conservation (i.e. less flow to the fetus) and paternally expressed genes in resource extraction (i.e. more energy to fetus) [8]. This provides a mechanism for the increasingly recognized paternal contribution to fetal growth disorders. The association between pre‐eclampsia and hypertensive disorders of pregnancy is well known. There is increasing evidence for the association of maternal haemodynamic changes, which may be subclinical, and fetal growth restriction. Reduced maternal cardiac output, lower heart rate and increased peripheral vascular resistance is seen in growth restriction and quite possibly pre‐date its development. There is also evidence of increased vascular stiffness and endothelial dysfunction. Whether these effects are a consequence of growth restriction or cause the condition remains to be elucidated. Routinely, symphysis–fundal height (SFH), the distance from the symphysis pubis to the uterine fundus, is measured at each midwife or antenatal attendance from 24 weeks’ gestation. As a guide, the SFH in centimetres is equal to the number of weeks of gestation plus or minus 2 cm. After 36 weeks, the acceptable difference increases to 3 cm. However, SFH has poor intra‐ and inter‐observer reproducibility. SFH is also prone to error due to factors unrelated to fetal biometry, such as maternal obesity, fibroids, polyhydramnios and fetal lie. There has been recent interest in standardizing SFH measurement by introducing training packages, and plotting the measurement on SFH charts that are generated at booking and adjusted for maternal characteristics. The validity and usefulness of this approach remains to be proven. Ultrasound is the current gold standard for fetal growth assessment. Measurements are made of the head circumference, abdominal circumference and femur length. In some cases, biparietal diameter may also be measured. These measurements are inputted into an algorithm, the most common being that developed by Hadlock, to calculate EFW, particularly the four‐parameter model. This can then be plotted against gestation‐specific centiles. There remains controversy as to whether fetal growth is the same in all healthy populations, or whether specific differences exist. Two recent studies, INTERGROWTH and WHO, both carried out in different centres throughout the world, have reached different conclusions on this matter. INTERGROWTH suggests that fetal growth is no different in optimally healthy women throughout the world, whereas the WHO study has shown small but significant differences in growth. The controversy is sure to continue, and with it the question of whether customizing birthweight percentiles based on maternal characteristics is appropriate. The assessment of the cause of an SGA fetus has important implications for the antenatal management and long‐term prognosis. At initial presentation, accuracy of dating of the pregnancy should be assessed and expected date of delivery confirmed. Gestation should be assigned by crown–rump length between 45 and 84 mm (11+2 to 13+6 weeks’ gestation); beyond this gestation, head circumference should be used, although in cases of in vitro fertilization (IVF), embryo transfer dates can be used with a correction for day 2 or 5 transfer. Evidence of poor placentation, failure of adaptation of the spiral arteries and adaptation of the maternal vasculature can be assessed by increased impedance and/or ‘notching’ in the uterine arteries (Fig. 17.1). Abnormal fetal Doppler indices or oligohydramnios are also suggestive of placental dysfunction as the cause of a small fetus. Fig. 17.1 (a) Normal and (b) abnormal uterine artery Doppler waveforms. The waveform in (b) has reduced diastolic flow velocities and an early diastolic notch. A small fetus may have an underlying chromosomal abnormality, particularly when presenting in the second trimester, and invasive testing should be discussed. A detailed anomaly scan should be performed, as any further anomaly would increase this risk further. Serological testing for cytomegalovirus and toxoplasmosis should be performed in severely growth‐restricted fetuses. It should be borne in mind, though, that features of placental dysfunction (see above) may also be seen in a poorly functioning placenta secondary to a chromosomal abnormality or infection. After exclusion of these causes, it should be considered whether a small fetus is constitutionally small. In such cases surveillance is warranted, although these fetuses will often follow a normal growth trajectory and have a good prognosis. Because of its heterogeneous aetiology, prediction of growth restriction is complex particularly in first pregnancies. At booking, which usually takes place at 10–13 weeks’ gestation, women can be assessed for risk factors. From history alone, these can be subdivided into maternal risk factors, maternal medical conditions and previous pregnancy history (Table 17.2). Paternal SGA should also be noted as this has an odds ratio (OR) of 3.7 for an SGA fetus. Table 17.2 Pre‐existing and maternal factors associated with fetal growth restriction. APH, antepartum haemorrhage; MoM, multiple of median; SGA, small for gestational age. As pregnancy progresses, further risk factors may become evident. In women undergoing combined screening for common trisomies, pregnancy‐associated plasma protein A (PAPP‐A) is routinely measured. PAPP‐A is a placentally derived protease whose main substrate is insulin‐like growth factor binding proteins and its serum levels are related to both the function and size of the placenta. There is an association between low PAPP‐A levels and low birthweight. Multiple other serum markers have been studied. These include those related to placental mass and function (e.g. ADAM‐12, PP‐13, IGFBP‐1 and IGFBP‐3), those related to angiogenesis (e.g. VEGF, endoglin, PGFa and sFlt‐1) and those related to endothelial function (e.g. asymmetrical dimethylarginine and homocysteine). None of these has been found to have predictive value that is of clinical use [9]. Ultrasound parameters have been shown to have some utility. The impedance to flow within the uterine arteries can be readily measured. In healthy pregnancy there is normally low resistance and high flow. Abnormalities of uterine artery Doppler waveform include increased impedance and ‘notching’ (Fig. 17.1). Uterine artery Doppler performed at 24 weeks’ gestation has 80% sensitivity for a 5% screen‐positive rate for the prediction of a fetus that will require delivery prior to 34 weeks due to growth restriction. The predictive value of uterine artery Doppler on its own in the first trimester has low sensitivity. Various algorithms have been developed for the prediction of fetal growth restriction. These commonly use a combination of maternal history, maternal physiological measurements, serum markers and ultrasound parameters. To date, none has had sufficient sensitivity at an acceptable false‐positive rate to warrant introduction into routine clinical practice. There is a clear association between growth restriction and hypertensive disorders of pregnancy, particularly pre‐eclampsia. Up to 70% of women with a growth‐restricted fetus will develop pre‐eclampsia. As such, blood pressure and urine dipstick for proteinuria should be performed at each visit. The umbilical artery Doppler waveform is the primary Doppler assessment in fetuses with growth restriction. Management protocols based on measurement of the umbilical artery waveform in high‐risk pregnancies has been shown to reduce perinatal deaths by up to 29%, though it is not clear on what basis decision‐making is improved. As placental dysfunction increases, there is increasing impedance in placental vessels. This initially presents as raised umbilical artery impedance, detected by increased values in either the resistance index (RI) or pulsatility index (PI). As the uteroplacental circulation is increasingly impaired, absent EDF in the umbilical artery is noted, indicative of loss of normal function in about 60–70% of villi, with progression to reversed EDF as remaining normal placental function is lost (Fig. 17.2). The progression of changes in umbilical artery Doppler waveforms can be rapid and unpredictable, particularly in early‐onset fetal growth restriction (FGR). Absent or reversed EDF after 26 weeks of gestation has been shown to have an independent impact on neurodevelopment. Fig. 17.2 Umbilical artery Doppler waveforms: (a) normal; (b) absent end‐diastolic flow; (c) reversed end‐diastolic flow. (See also colour plate 17.2) A recent Cochrane systematic review and meta‐analysis, including 18 studies and over 10 000 women, demonstrated that women who had Doppler assessment had a significantly lower perinatal mortality (1.2%) compared with those who had not been assessed by Doppler (1.7%; relative risk or RR 0.67; 95% CI 0.46–0.96). Although the data for secondary outcomes showed that there were fewer adverse outcomes in the Doppler group, this did not reach statistical significance [10]. Interestingly, there was a reduction in interventions including induction of labour and lower segment caesarean section in the Doppler group. There was no difference in operative vaginal delivery rates or Apgar scores at 5 min. Notably, though, there is a lack of data on long‐term neurological development in the babies in either group. Unlike umbilical artery Doppler, middle cerebral artery (MCA) Doppler can be a proxy for hypoxia and may be abnormal for many weeks in early‐onset FGR. The role of the cerebral arteries and the changes that occur in the vessels are important in relation to the concept of ‘brain‐sparing’ in the chronically, or indeed acutely, hypoxic fetus. Although now debated, the concept of ‘brain‐sparing’ involves redistribution of blood by dilatation of the cerebral vessels, thus increasing substrate and oxygen supply to the brain, in response to fetal chemoreceptor or baroreceptor stimulation. The value of MCA Doppler in the prediction of adverse fetal outcome and assessment of the at‐risk fetus has been reported variably. Some studies have suggested that assessment of MCA Doppler is a useful tool, whereas others have found poor predictive value [11–14]. Recently, a meta‐analysis of eligible studies (35 in total) including 4025 fetuses has been performed [15]. This found that low MCA PI appears to be associated with fetal well‐being assessed by acidosis (pH <7.20) at birth (although this finding is largely biased by a single study in a high‐risk population [16]), Apgar score below 7 at 5 min or admission to neonatal intensive care. It is important to recognize that while these findings do suggest that there is an association between abnormal MCA and adverse outcomes, the association is weak. Overall, this meta‐analysis suggests that in clinical practice MCA alone has limited predictive value for compromise of fetal or neonatal well‐being. As discussed in the previous section, the normal physiological response of MCA dilatation in fetuses exposed to acute or chronic hypoxic conditions results in a reduction in fetal MCA PI. Commonly, though not universally, this is associated with increasing impedance in the umbilical artery. Whilst the values obtained on measuring these Doppler parameters individually may be within normal limits, it is possible that the fetus is compensating. By using the ratio of MCA to umbilical artery Doppler PI – the cerebroplacental ratio (CPR, also called the cerebro‐umbilical ratio) – it may possible to determine which fetuses may be at risk of compromise, as those with abnormal (i.e. low) CPR may be considered to have failed to reach their growth potential [17,18]. A low CPR [17,19] may be associated with delivery by lower segment caesarean section due to fetal compromise, determined by either abnormal CTG or fetal blood sample below pH 7.20, although no differences in Apgar scores and arterial pH (taken at time of delivery) were found nor is there evidence of adverse neonatal outcomes in the groups with low CPR in either study. However, others [20] have also associated low CPR (PI <1) with adverse perinatal outcome in intrauterine growth restriction (IUGR) and impaired neurodevelopment at 2 years of age. CPR may be of most use when umbilical artery PI is greater than the 95th centile with a normal waveform, in which situation a low CPR gives an OR for adverse perinatal outcome of 11.7 (95% CI 6.0–22.9). This provides similar predictive value to an abnormal umbilical artery waveform (OR 10.8, 95% CI 3.8–30.5) compared with raised umbilical artery PI alone (OR 6.9, 95% CI 2.9–16.5). It should be remembered that gestation and birthweight remain the most important predictors of morbidity and long‐term outcome. The ductus venosus (DV) is a fetal vessel connecting the intra‐abdominal portion of the umbilical vein to the left portion of the inferior vena cava just below the diaphragm [21]. The function of the DV is to shunt the oxygen and substrate‐rich blood coming from the placenta via the umbilical vein to the heart. The DV diverts 25% of the blood to the heart, with the remainder being distributed to the liver and joining the circulation via the hepatic portal system. Although entering the heart via the right atrium, this substrate‐ and oxygen‐rich blood is preferentially directed to the left atrium and then, via the left ventricle and aorta, to the fetal heart and brain [22,23]. The utility of the DV waveform is primarily in the very premature fetus with IUGR, or the preterm fetus with abnormal umbilical artery waveforms (Fig. 17.3). Reversal or absence of the DV a‐wave, particularly in combination with umbilical vein pulsations, has been shown to be closely associated with a pH below 7.20 [24] and perinatal mortality irrespective of gestational age, with a risk of up to 100% in early‐onset FGR. It has been shown that DV abnormalities precede changes in computerized CTG in 50% of cases, although it is safe to wait DV PI or waveform changes in cases where computerized CTG remains normal [25]. Fig. 17.3 Ductus venosus waveforms: (a) normal; (b) reversed a‐wave. (See also colour plate 17.3) The TRUFFLE study and the application of its results to the timing of delivery in early‐onset IUGR is discussed later. CTG monitoring as an assessment of fetal well‐being has become a routine part of antenatal care. There is, however, no evidence of benefit in high‐risk cases and may in fact result in unnecessary intervention [26]. However, computerized CTG monitoring can provide assessment of short‐term variability (STV), which cannot be performed by visual assessment alone. Reduced STV (<3 ms) has been shown to correlate with the fetal metabolic state and significantly reduced STV is closely associated with fetal acidaemia [27–29]. This has been shown to reduce perinatal mortality compared with traditional CTG in the high‐risk population (RR 0.20, 95% CI 0.04–0.88) [26], although this effect was not evident once deaths due to congenital anomalies were excluded (OR 0.23, 95% CI 0.04–1.29). As such, CTG monitoring should not be used as the only form of surveillance in SGA fetuses. If CTG monitoring is used, interpretation should be based on analysis of STV on computerized CTG [30]. The biophysical profile combines the use of CTG with the ultrasound assessment of fetal movement, fetal tone, fetal breathing movements and amniotic fluid. Each parameter is scored 0–2 points, with a maximum total score of 10. A normal score (8 or greater) is reassuring. Biophysical profile has a high false‐negative rate and is not a good predictor of fetal acidaemia. It has also been shown to increase caesarean section rates, but not improve perinatal outcome. The use of biophysical profile is not recommended for surveillance of the SGA fetus. Timing of delivery of the small or growth‐restricted fetus can be challenging. This is particularly so at very preterm gestations, which are associated with high rates of perinatal complications. Neonatal morbidity and mortality have improved significantly in the past decade, with survival rates of 13% at 24 weeks, 43% at 25 weeks, up to 76% at 26 weeks and up to 90% at 30 weeks’ gestation [31]. Timing of delivery must take into account not only survival but also associated significant morbidities such as bronchopulmonary dysplasia, intraventricular haemorrhage, necrotizing enterocolitis and cerebral palsy. Similarly, neonatal survival without significant morbidity progressively increases with increasing gestational age. However, both birthweight centile and gestational age are important factors at very preterm gestations and must be considered in combination when considering neonatal outcomes. The PREM scoring system has also been recently validated [32] and it has been suggested that it might be used in combination with the Clinical Risk Index for Babies (CRIB) scoring system for neonatal mortality risk adjustment. Outcomes for growth‐restricted neonates below 29 weeks are similar to those of appropriately grown neonates delivering at a gestation 2 weeks earlier [31]. Below 32 weeks, timing of delivery can be based on DV waveforms or computerized CTG analysis of the STV. The TRUFFLE study randomly assigned growth‐restricted fetuses between 26 and 32 weeks’ gestation to delivery based on one of DV PI above the 95th centile, absent DV a‐wave or abnormal STV [33]. The results showed that neonatal survival without neuroimpairment was similar across all groups. However, among survivors at 2 years there were better functional outcomes in those delivered based on waiting for late DV changes rather than CTG abnormality. The earlier GRIT study had shown no difference in outcomes at 2 years between immediate delivery and delayed delivery in compromised fetuses between 24 and 36 weeks’ gestation. Based on the fact that these survival rates are good, with low morbidity rates beyond 32 weeks [34,35] and, in light of TRUFFLE data, appear to be improving, delivery of an SGA fetus with absent or reversed EDF in the umbilical artery should be recommended at 32 weeks’ gestation. Beyond 36 weeks, delivery may be informed by the findings of the Disproportionate Intrauterine Growth Intervention Study at Term (DIGITAT) study. This study randomized women between 36+0 and 41+0 weeks’ gestation with fetuses less than the 10th centile to either delivery within 48 hours or expectant management. There were no significant differences in long‐term outcomes between the two management strategies [36]. An increased incidence of admission to neonatal care was found in the delivery group [37]. Importantly, there was a higher perinatal mortality in women who did not consent to take part in the study and who in the main opted for conservative management, i.e. waiting, without a clear protocol for monitoring. In the absence of harm, SGA fetuses beyond 37 weeks can be considered for delivery and delivery should be recommended if there are Doppler abnormalities, including increased umbilical artery PI or decreased MCA PI. However, a pragmatic approach to delay delivery beyond 38+0, if clinically possible, may reduce neonatal admissions. There remains an absence of high‐quality data to guide the timing of delivery between 32 and 36 weeks’ gestation. A multicentre European study (TRUFFLE 2) is currently in development to address this. An approach of conservative management with increased surveillance with Doppler and CTG can be adopted in these cases. At 37 weeks, delivery should be considered, as outlined above. Prior to this, if there are any clinical concerns, Doppler waveform abnormalities or reduction in growth velocity, earlier delivery should be advised.
Fetal Growth Restriction
Definition
Early‐onset growth restriction
AC or EFW <3rd centile or absent EDF in the umbilical artery
or
Late‐onset growth restriction
AC or EFW <3rd centile
or
At least two of three of the following:
Consequences of growth restriction
Regulation of fetal growth
Assessment and investigation
Symphysis–fundal height
Ultrasound biometry
Customized charts
Causes
Prediction
Risk
OR (95% CI)
Pre‐existing maternal factors
Age >40 years
3.2 (1.9–5.4)
Smoker >11/day
3.21 (2.03–2.4)
Cocaine use
3.23 (2.43–4.3)
Vigorous exercise
3.3 (1.5–72)
Maternal obstetric history
Previous SGA
3.9 (2.14–7.12)
Previous stillbirth
6.4 (0.78–52.56)
Maternal SGA
2.64 (2.28–3.05)
Maternal disease
Chronic hypertension
2.5 (2.1–2.9)
Diabetes with vascular disease
6 (1.5–2.3)
Renal impairment
5.3 (2.8–10)
Antiphospholipid syndrome
6.2 (3.47–10.27)
Paternal factors
Paternal SGA
3.47 (1.17–5.6)
Pregnancy complications
Heavy bleeding
2.6 (1.2–5.6)
Pregnancy‐induced hypertension
2.5 (2.3–2.8)
Pre‐eclampsia
2.26 (1.22–4.18)
Echogenic bowel
2.1 (1.5–2.9)
Unexplained APH
5.6 (2.5–12.2)
PAPP‐A <0.4 MoM
2.6
Low weight gain
4.9 (1.9–12.6)
Monitoring SGA
Umbilical artery Doppler
Middle cerebral artery Doppler
Cerebroplacental ratio
Ductus venosus Doppler
Cardiotocogram and biophysical profile
Timing of delivery
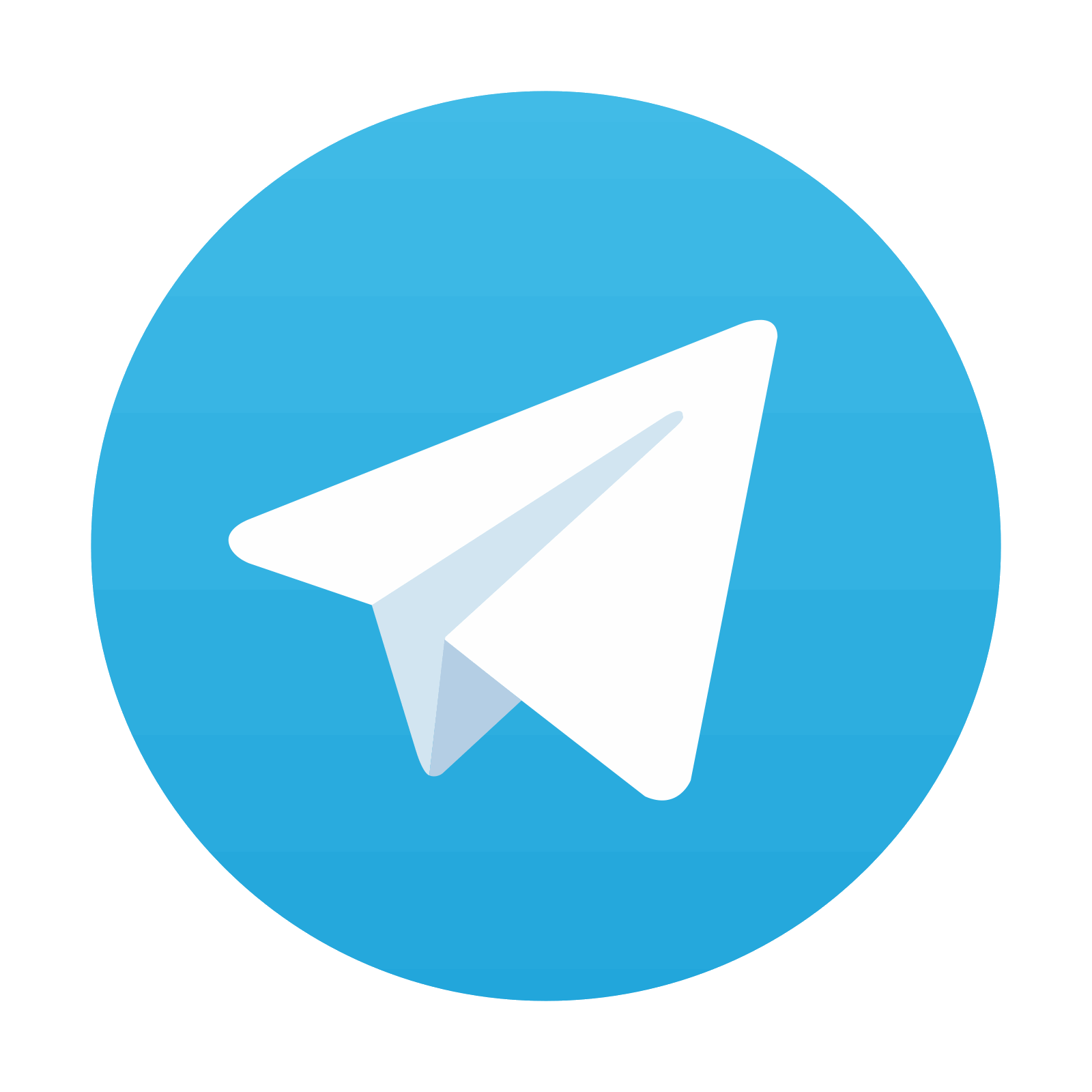
Stay updated, free articles. Join our Telegram channel
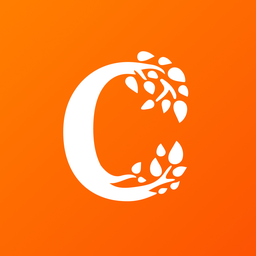
Full access? Get Clinical Tree
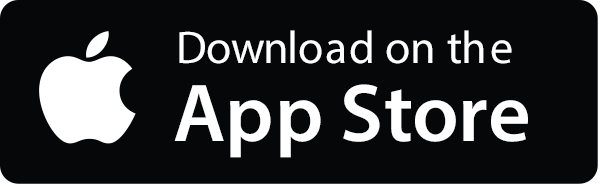
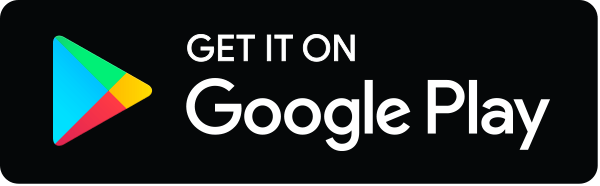