CHAPTER 19 Fertilization to implantation
Introduction
At fertilization, two gametes fuse to generate an embryo which subsequently initiates an elaborate developmental programme whilst negotiating its way to the uterine cavity, the site of implantation. Recent years have seen great strides in our understanding of the molecular minutiae underpinning these events. These advances have furthered insight into human reproductive failure, guided the refinement of treatment designed to alleviate subfertility, and contributed to the evolution of avant-garde scientific developments, most notably stem cell technology.
This chapter provides an overview of fertilization, preimplantation embryonic development and implantation, and their collaboration in reproduction. The chapter will begin by outlining the essential prerequisites for fertilization, and conclude with a bird’s eye view of some of the clinical and scientific innovations that have been mined from an improved understanding of these elaborate processes.
Prelude to Fertilization
Sexual dimorphism in gametogenesis
Male gametes (spermatozoa) and female gametes (ova or eggs) possess half the normal chromosome complement. The merging of two gametes at fertilization restores the complete chromosome set in the embryo.
Spermatogenesis and oogenesis produce male and female gametes, respectively, and are characterized by a unique nuclear division, known as ‘meiosis’, which halves chromosome numbers. Notably however, the tempo of gametogenesis differs markedly between males and females. Spermatogenesis progresses uninterruptedly over approximately 60–70 days, whereas oogenesis often spans decades and is a discontinuous process punctuated by two arrest stages (Homer 2007).
With the exception of the relatively small amount of DNA associated with mitochondria which is exclusively oocyte-derived, spermatozoa and eggs have comparable stakes in the embryo’s genetic blueprint. In stark contrast, male and female gametes make very different cytoplasmic contributions (Figure 19.1). Virtually all of the embryo’s biosynthetic raw materials and organelles are derived from the egg, with the centriole being the only male cytoplasmic contribution. The fate of an embryo is therefore critically dependent upon stores built up in the oocyte during an extended growth phase lasting 2–3 months, resulting in a 100-fold increase in oocyte volume (Telfer and McLaughlin 2007). Consequently, any compromise to egg quality, such as is seen with advancing female age, has devastating consequences for embryonic and reproductive potential (Homer 2007). Accordingly, older women experience lower in-vitro fertilization (IVF) success rates, along with increased risks for miscarriage and chromosomally abnormal offspring.
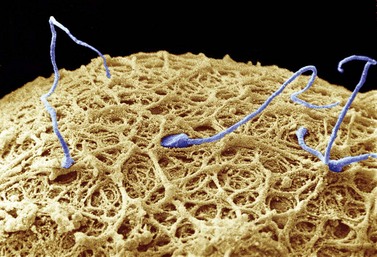
Figure 19.1 Colour-enhanced scanning electron micrograph of human sperm attempting to fertilize an egg. Note the marked difference in size between the spermatozoa and the egg.
Reproduced with permission from the Wellcome Trust.
In stark contrast to oogenesis which has embryonic sustenance as an over-riding concern, spermatogenesis aims to produce a lightweight and motile gamete capable of penetrating the oocyte. Spermatogenesis fulfils this objective by incorporating a remodelling phase known as ‘spermiogenesis’, the most remarkable cellular metamorphosis in the human body. Spermiogenesis strips the spermatid of almost all of its cytoplasm, makes the nucleus highly compact by integrating unique DNA-binding proteins called ‘protamines’ (Sassone-Corsi 2002), and fashions a propulsive tail with an adjacent fuel source in the mitochondria-laden mid-piece (Figures 19.2 and 19.3). A specialized lysosomal-like secretory granule known as the acrosome is also forged, forming a cap-like structure over the nuclear surface (Figures 19.2 and 19.3). The acrosome contains enzymes essential for digesting a path through the egg’s outer covering known as the ‘zona pellucida’.
Maturation and capacitation
On release into the seminiferous tubule lumen, spermatozoa are immotile and lack fertilizing capacity, only acquiring these facets after maturation and capacitation.
Maturation occurs in the epididymis endowing spermatozoa with the potential for movement and fertilization. The molecular basis for maturation remains unresolved, but is known to depend upon androgens and exposure to the dynamic microenvironment created by the secretory and absorptive functions of the epididymal epithelium (Nixon et al 2007).
Capacitation enables spermatozoa to recognize their full fertilization potential and occurs within the female genital tract. It is characterized by cyclic adenosine monophosphate (cAMP)-dependent tyrosine phosphorylation of intracellular proteins, most of which are components of the sperm exocytotic and motility machinery (Nixon et al 2007). The capacitated spermatozoon is now distinguished by hyperactivated motility and a change in surface membrane properties, rendering it sensitive to signals encountered in the immediate vicinity of the ovulated egg.
Transport of gametes in the female genital tract
At coitus, sperm are deposited in the upper vagina and on the external cervical surface. In spite of coagulum formation, more than 99% of sperm are lost by leakage from the vagina, with only a small minority gaining access to the relative haven of the cervical crypts. From there, only about one in every million spermatozoa will successfully navigate the 16–20-cm journey to the tubal ampulla, propelled by innate motility and aided by currents created by tubal ciliary activity.
At the time of ovulation, the egg and its tunic of cumulus cells are directed by tubal fimbria and cilia into the tubal ostium and then towards the tubal ampulla (Figure 19.4). The cumulus–egg complex attracts sperm by releasing chemoattractants that stimulate sperm odorant receptors, thereby inducing cAMP-mediated sperm chemotaxis.
Fertilization
Fertilization commences with spermatozoal penetration of the oocyte’s outer coverings, and concludes with syngamy, the amalgamation of male and female paternal genomes on the first mitotic spindle, altogether lasting approximately 18–21 h.
Cumulus penetration
The first obstacle encountered by any would-be suitor of the ovulated egg is a covering of cumulus cells embedded in an extracellular matrix comprised primarily of the glycosaminoglycan, hyaluronan (Figure 19.5A). Spermatozoa digest a path through the extracellular matrix via hyperactivated motility and a glycophosphatidyl inositol (GPI)-anchored surface hyaluronidase termed ‘PH-20’ (Primakoff and Myles 2002). Notably, eggs influence sperm penetration, albeit indirectly, by secreting factors such as growth differentiation factor-9 and bone morphogenic protein-15 which regulate cumulus integrity (Swain and Pool 2008).
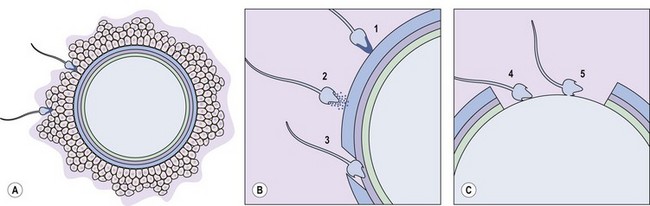
Figure 19.5 Stages of fertilization. (A) Acrosome intact spermatozoa penetrate the cumulus cells to reach the zona pellucida. (B) Spermatozoa then bind to the zona (1), undergo the acrosome reaction (2) and penetrate the zona (3) before entering the perivitelline space. (C) In the perivitelline space, the equatorial head region of the spermatozoa binds the egg plasma membrane (4), after which fusion occurs (5).
From Primakoff and Myles, Penetration, Adhesion, and Fusion in Mammalian Sperm-Egg Interaction, Science; 296; 2183–2185; 2002, Reprinted with permission from AAAS.
Sperm–zona interaction
After cumulus penetration, sperm must engage and penetrate the zona pellucida (Figure 19.5B and C). The zona is secreted by the growing oocyte and, in humans, comprises four glycoproteins: ZP1, ZP2, ZP3 and ZP4 (Wassarman 2008). ZP3 in concert with O-linked oligosaccharides functions as the primary sperm receptor that preferentially binds the plasma membrane overlying the intact acrosome. ZP2 is postulated to serve as a secondary receptor that attaches to the inner acrosomal membrane of acrosome-reacted sperm. The zona is also important for mediating species specificity in gamete interaction, and for preventing polyspermy following the cortical reaction (discussed later).
The complementary spermatozoal ligand for ZP3 defies unequivocal identification at present. It appears that the coordinated action of several proteins such as GalTI-1 (β-1,4-galactosyltransferase) and SED1 that are recruited and constrained by lipid rafts on the sperm surface may mediate docking of sperm on to the zona (Nixon et al 2007).
Binding of sperm ligands with ZP3 induces a rise in intracellular calcium, triggering multiple sites of fusion between the sperm membrane and the outer acrosomal membrane (Figure 19.6). This culminates in exocytosis of sperm acrosome contents, the acrosome reaction (Figures 19.5B and 19.6). The proteases and glycosidases thus released, in concert with hyperactivated motility, enable sperm to digest a path through the zona (Figure 19.5B).
Sperm–egg binding and fusion
Having penetrated the zona, spermatozoa enter the perivitelline space between the egg membrane (oolemma) and the zona (Figure 19.5B). Here, further receptor–ligand interactions enable binding of equatorial sperm membrane and oolemma, followed by insertion of the sperm membrane into the opposing oocyte bilayer at fusion (Figure 19.5C).
Initial interest in interplay between oolemmal integrins (e.g. α6β1) and sperm disintegrins (e.g. fertilin and cyritestin) as mediators of binding-fusion have dissipated due to lack of corroborative evidence from integrin/disintegrin knockout mouse models. Instead, focus is currently on alternative candidates such as sperm lysozyme-like protein and Izumo on sperm, and GPI-anchored proteins and the CD9 tetraspanin on eggs (Kaji et al 2000, Nixon et al 2007).
Egg activation
Sperm–egg fusion induces calcium oscillations in the egg emanating from the point of sperm entry. These calcium transients result from inositol triphosphate production brought about by a soluble sperm-specific factor known as ‘phospholipase-Cζ’ (Swann et al 2006). Calcium signals induce the two principal events associated with egg activation: breaking the second meiotic arrest and the cortical reaction.
At the time of fusion, the egg is arrested in meiosis II. This state of suspended animation is dependent upon maturation-promoting factor that is itself sustained by an ooplasmic activity known as ‘cytostatic factor’ (Tunquist and Maller 2003). The recent characterization of two proteins, early mitotic inhibitor 2 and the calcineurin phosphatase, has elucidated the link between calcium and meiosis II resumption. Following fusion, the ensuing calcium surge induces calcium/calmodulin-dependent-kinase-II-dependent early mitotic inhibitor 2 destruction and transient calcineurin activation, together resulting in cytostatic factor and maturation-promoting factor inactivation and relief from meiosis II arrest (Mochida and Hunt 2007, Wu and Kornbluth 2008).
Formation of pronuclei and syngamy
Following fusion, the sperm nucleus enters the ooplasm accompanied by its centriole and flagellum. The male centriole is required for nucleating spindle microtubules and thus for cell division, the female equivalent having been lost during the oocyte’s growth stage. On entering the ooplasm, sperm nuclear membranes dissolve and the highly condensed male chromatin undergoes decondensation as its constituent protamines are reduced by glutathione and ultimately replaced by histones present in the ooplasm.
Pronuclei formation in the resulting zygote involves the reformation of a nuclear envelope around each of the haploid parental genomes. The pronuclei migrate towards one another and their constituent chromosomes undergo replication in preparation for the first mitotic division of the embryo. Following DNA duplication, pronuclear membranes break down, and the maternal and paternal chromosomes come together for the first time on the first mitotic spindle at syngamy. Immediately thereafter, chromosomes move apart at anaphase, the cleavage furrow forms and the one-cell zygote becomes a two-cell embryo.
Preimplantation
Preimplantation development encompasses the period from fertilization to implantation. Key events occurring during this time include continued cell division, morphological refashioning and genetic reprogramming.
Timelines and key morphologic changes of embryonic development
During early embryonic divisions, increasing cell number is not accompanied by any appreciable increase in embryonic size, reflected by the continued presence of the zona (Figure 19.7). Instead, during this time, the cytoplasm inherited from the egg is merely redistributed to the accumulating blastomeres which get progressively smaller, again vividly illustrating the central role played by the egg in embryogenesis.
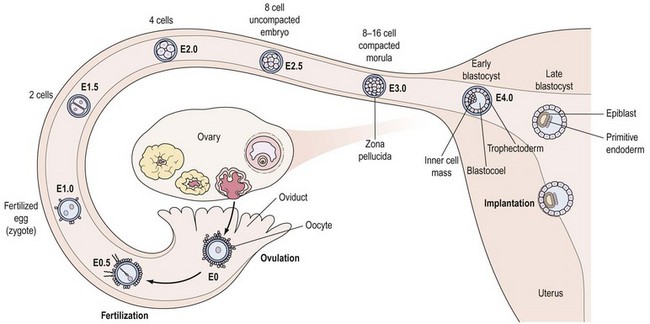
Figure 19.7 Timeline of preimplantation embryonic development during transit of the embryo through the fallopian tube and into the uterine cavity.
From Wang and Dey; Roadmap to embryo implantation: clues from mouse models; Nature Reviews Genetics, 7;185–199,2006.
Around the eight-cell stage (3–4 days post ovulation), the embryo undergoes compaction whereby membrane surfaces between neighbouring embryonic cells (or blastomeres) become indistinct, leading to the formation of a morula by the 16-cell stage. Around this time, the embryo enters the uterine cavity to begin its rapport with the endometrium (Figure 19.7). Shortly thereafter, at the 32–64-cell stage (4–5 days post ovulation), the blastocyst is formed, characterized by the presence of a cavity or blastocoel and the presence of two cell types: an outer trophoectoderm (TE) or trophoblast which is the placental precursor, and an eccentrically placed inner cell mass (ICM) which is the progenitor of the embryo proper (Figure 19.7). As discussed in greater detail later, isolation and culture of cells from the inner cell mass at this stage results in the derivation of an embryonic stem (ES) cell line (see Figure 19.10C).
Oviductal transport and nutritional support
Tubal embryo transport is dependent upon coordinated oviductal smooth muscle activity mediated by the sympathetic nervous system. The emerging picture is that sympathetic tone requires fine-tuning by lipid mediators, known as ‘endocannabinoids’ (e.g. anandamide), acting via CB1 receptors. Human fallopian tubes were recently shown to express CB1, the levels of which peak during the luteal phase, likely reflecting control by progesterone (Horne et al 2008). The importance of endocannabinoid signalling is underlined by high rates of oviductal embryo retention in CB1-deficient mice (Wang et al 2004), and lowered tubal CB1 expression in women with ectopic pregnancies (Horne et al 2008).
The embryo’s nutritional requirements change during early development. Thus, the early-cleavage-stage embryo is relatively metabolically quiescent and utilizes pyruvate as an energy source, whereas blastocysts demonstrate high metabolic activity and use glucose as their sole energy supply. In response to this, the oviduct is rich in pyruvate and non-essential amino acids, and low in glucose, whereas the uterine cavity is rich in glucose and essential amino acids (Watkins et al 2008).
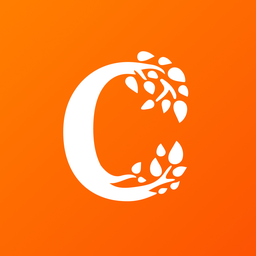
Full access? Get Clinical Tree
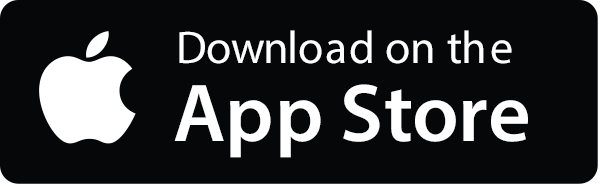
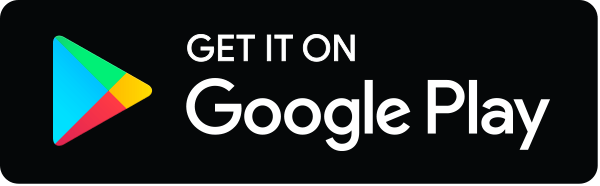