Fig. 4.1
Fertilization process. 1 Sperm penetration of cumulus cells, 2 attachment to zona, 3 exocytosis of acrosomal contents, 4 penetration to the zona pellucida, 5 entry into perivitelline space, 6 binding and fusion with the egg plasma membrane, 7 cortical reaction, and 8 block to polyspermy. Reproduced with permission from Esfandiari N. In: Hurd WW, Falcone T, eds. Clinical reproductive medicine and surgery. St. Louis, MO: Mosby/Elsevier; 2007
4.9 Structure of Zona Pellucida and Sperm Penetration
Once spermatozoa pass through the cumulus oophorus, they bind zona pellucida, which is the thick extracellular coat of the egg. (◘ Fig. 4.2) [57]. The sperm to zona pellucida binding is a species-specific process. This concept is the basis of the “hamster zona binding test.” Human sperm cannot bind to hamster eggs with an intact zona pellucida, which led to the thought that the zona pellucida contains species-specific receptors. Human sperm can only bind to hamster eggs after this glycoprotein layer is removed. Although there are some species–species exceptions, the zona pellucida is an important barrier between interspecies fertilization. In the clinical setting, the sperm penetration assay, the sperm–zona pellucida binding, the acrosome reaction, and the hyaluronan binding can be utilized in workup of subfertile men [58].

Fig. 4.2
Light microscopy of mouse sperm binding the zona pellucida of an unfertilized egg. (Source: Wassarman PM, Jovine L, Litscher ES. A profile of fertilization in mammals. Nat Cell Biol. 2001 Feb;3(2):E59–64. Used with permission from Nature Publishing Group)
With the aid of electron microscopy and advanced molecular techniques, our understanding of the zonal structure has increased. There are three major glycoproteins that compose the zona pellucida and have distinct roles in this structure: ZP1, ZP2, and ZP3 [59]. The ZP2 and ZP3 proteins form a filamentous structure that is then cross-linked with ZP1 proteins [60, 61].
In a classical model, ZP3 binds sperm and initiates the acrosomal reaction (see ◘ Fig. 4.1). Mutagenesis of O-glycosylation sites of ZP3 has been shown to decrease sperm receptor activity, suggesting that ZP3 serves as the sperm receptor in zona pellucida [62]. As sperm binds to ZP3, the outer acrosomal membrane fuses with the sperm plasma membrane that subsequently causes membrane blebs and results in the releasing of acrosomal enzymes that lyse the zona pellucida. This reaction exposes the inner acrosomal membrane that can bind to ZP2 (◘ Fig. 4.3) [63]. Eventually, sperm penetrate the zona pellucida and enter into the perivitelline space. Various other models have shown that this acrosomal reaction could occur when sperm encounter cumulus cells [64]. However, as mentioned above, some oocytes do not have cumulus cells and can still be fertilized.

Fig. 4.3
Sperm and ZP3 binding. Acrosome intact spermatozoon shown with red crescent on its head, whereas acrosome reacted spermatozoon does not. A- ZP3 binds spermatozoon and induces acrosome reaction, thereby releasing of acrosomal enzymes that lyse the zona pellucida. Acrosome-reacted spermatozoon binds ZP2 via their exposed inner acrosomal membrane and penetrate the zona pellucida, ultimately fusing with the oocyte. B- Immediately after fertilization cortical granules release proteases to the perivitelline space that clip ZP2 and converts it to cleaved ZP2 (ZP2c) that can no longer bind acrosome-reacted sperm. Cleaved ZP2 dissociates from ZP3, resulting in subtle modification of ZP3 to convert it ZP3f that lacks sperm receptor and acrosome inducing capability. (Source: Clark GF, Reproduction. 2011 Sep.; 142(3):377–81. Used with permission from Bioscientifica Ltd.)
4.10 Cortical Reaction to Block Polyspermy
As the spermatozoa enter the perivitelline space, they initiate the cortical reaction (see ◘ Fig. 4.1). Release of proteolytic enzymes from egg cortical granules causes cleavage of ZP2, with subsequent dissociation of ZP2 from ZP3 [65]. Thus, after the cortical reaction, sperm can no longer bind to ZP3 (◘ Fig. 4.3) [63]. Additionally, cleaved ZP2 cannot bind a spermatozoon that had previously undergone an acrosomal reaction. In conclusion, neither a sperm with an intact acrosome nor a sperm that has undergone an acrosomal reaction would be able to bind to the zona pellucida after the cortical reaction [63]. This is the principal mechanism preventing polyspermy.
Although through murine models we have learned an impressive amount about the process of fertilization, there are still many questions that need to be answered and further research on the exact mechanism of sperm binding is needed.
4.11 Sperm-Oocyte Membrane Fusion
After a spermatozoon penetrates the zona pellucida and enters the perivitelline space, the oocyte membrane and the spermatozoon membrane unite (see ◘ Fig. 4.1). At this stage, the spermatozoon has already undergone an acrosomal reaction, which exposed the inner acrosomal membrane and modified the membrane composition of both equatorial and post-acrosomal regions of the spermatozoon. The fertilizing spermatozoon binds to the microvillar region of the oocyte membrane with its equatorial segment [66]. Sperm tail movement decreases or stops within a few seconds of sperm-oocyte fusion [67]. Subsequently, the posterior region of the sperm head and the tail are incorporated into the egg. Unfortunately, the details of molecular interactions in sperm-egg fusion are not fully known. Initially, ADAM family members that are found on the sperm membrane, specifically fertilin and cyritestin, gained much attention. However, gene knockout studies questioned their fundamental roles in sperm-egg fusion. Currently, cyritestin, fertilin α(alpha), fertilin β(beta), CRISP1, izumo proteins, α(alpha)6β(beta)1 integrin, GPI-anchored proteins, CD151, CD9, and CD81 on the plasma membrane are thought to be involved in sperm-oocyte membrane fusion and are the subjects of ongoing research [68, 69] (◘ Fig. 4.4).

Fig. 4.4
Model for molecular interactions during sperm-egg binding. GPI-anchored proteins, integrins, CD151, CD9, CD81 on the oocyte membrane and ADAM proteins, Pdi3a chaperone refolding Izumo on the sperm membrane are involved in sperm-oocyte membrane fusion. (Source: Nixon B, Aitken RJ, McLauglin EA. Cell Mol Life Sci. 2007 Jul;64(14):1805–23. Used with permission from Springer)
4.12 Oocyte Activation
Mammalian oocytes become arrested at the metaphase of the second meiotic division. After sperm-oocyte fusion, the oocyte continues meiotic division, releases cortical granules, progresses cell cycle, forms its pronucleus, and recruits maternal mRNA that are all essential for gamete formation [70]. These morphologic and biochemical changes that occur in the oocyte are collectively called “oocyte activation.” Another important hallmark of oocyte activation is calcium oscillations. It has been shown that injecting calcium into mice oocytes is enough to trigger embryo development up to the blastocyst stage [71]. In a mammalian oocyte, the calcium oscillations are a direct result of inositol triphosphate-mediated calcium release. Sperm-derived phospholipase-zeta (PLC-ζ(zeta)) is also responsible for oocyte activation [72]. Another protein that has been shown to activate oocytes is post-acrosomal sheath WW domain-binding protein. Its exact signaling mechanism is not clearly known, but it presumably acts through calcium signaling [73]. Regardless of the signaling pathway, oocyte activation is essential for pronucleus formation and subsequent embryo formation.
Oocyte activation clearly has clinical importance. A deficiency in oocyte activation was regarded as the principal cause of fertilization failure or low fertilization rate after ICSI. Recently it has been suggested that PLC-ζ(zeta) could be used as an alternative oocyte-activating agent, including male factor infertility, similar to other artificial oocyte activators [74, 75].
4.13 Male Pronucleus Formation and Genomic Union
The final step of fertilization is the union of sperm and egg pronuclei, producing a diploid cell, the zygote. Dynactin, nucleoporins, vimentin, dynein, and microtubules are involved in bringing the two pronuclei together. It was proposed that a nuclear pore complex is inserted into the nuclear envelopes of the newly forming pronuclei. Dynactin and vimentin filaments are then incorporated into this nuclear pore complex. Formation of the complex probably starts after egg activation. The sperm aster then extends the microtubule “plus ends” away from the male pronucleus, some of which reach the female pronuclear envelope. With the aid of the dynactin-dynein motor complex, the two pronuclei are apposed [76]. Subsequently, the two nuclear envelopes disappear and the DNA undergoes replication. Homologous chromosomes are paired and aligned on the newly formed mitotic spindle. Eventually, the zygote is ready to undergo its first mitotic division.
4.14 Early Embryonic Development
In mammals, the zygote undergoes mitotic division (known as cleavage) as it travels through the fallopian tube, and eventually develops into a blastocyst once in the uterus (◘ Fig. 4.5). The symmetrical cell divisions and cleavage create a ball of totipotent cells (blastomeres) that are still enclosed in the zone pellucida. When the zygote is approximately 16 cells, blastomeres form a closely packed group of cells with a smooth outer surface. This early developmental event is called compaction. The smooth surface is created by the formation of adherens and tight junctions between the blastomeres. At this time, two types of polarity originate in the zygote. The first type of polarity is cellular polarity. Cellular polarity occurs as the formation of microvilli on the external surface of the outer blastomeres separate from the basolateral surface [77].The second type of polarity is developmental polarity. Developmental polarity is represented by the ability of the blastomeres in the internal compartment, the inner cell mass, to remain pluripotent, whereas the outer blastomeres begin to form trophoblast cells as they continue to divide [78]. This begins formation of the blastocyst and typically occurs around day 5 of fertilization. As cleavage continues, outer blastomeres express tight junction proteins, including ZO-1 and uvomorulin, gap junction proteins such as Connexin-43, and differentially position Na-K ATPase pumps selectively along the apical-basolateral axis. The outer blastomeres have a highly restricted developmental fate, eventually becoming the cells of trophoectoderm . The polarized expression of Na-K-ATPase in trophoectoderm creates a trans-trophoectoderm sodium gradient, which drives the osmotic accumulation of water into the nascent blastocoelic cavity. Growth factors like TGF-α(alpha) and EGF increase expression of Na-K-ATPase, which subsequently stimulate further expansion of the blastocoelic cavity (blastocoel). Meanwhile, the inner blastomeres continue to divide, and with the expansion of the blastocoel, they create a cluster of cells that impend into blastocoel . This totipotent cell cluster is commonly called the inner cell mass. The inner cell mass will eventually give rise to the embryo and extraembryonic tissues . The outer layer of blastomeres, which have developed into trophoblasts/trophoectoderm, eventually give rise to the placenta. It is at this point that the developing embryo is called a “blastocyst.”

Fig. 4.5
Schematic drawing showing the major events from ovulation to the implantation of blastocyst during the first week of human life. Reproduced with permission from Esfandiari N. In: Hurd WW, Falcone T, eds. Clinical reproductive medicine and surgery. St. Louis, MO: Mosby/Elsevier; 2007
Although difficult to completely exclude, this initial exponential division and formation of the blastocyst seems to be relatively independent of maternal contribution.
Around day 6 after ovulation, the embryo/blastocyst reaches the uterine cavity and is initially still covered with zona pellucida. For proper implantation, it must shed the zona pellucida. Trophoblast-derived trypsine-like enzymes, strypsin and plasmin, are thought to lyse the zona pellucida, allowing the embryo to hatch from the zona pellucida and begin to attach to the uterine endometrium [79, 80].
4.15 Trophoblastic Development and Invasion
The blastocyst is lined with a layer of trophoectoderm , which, as stated above, will give rise to the placenta. Although the inner cell mass is destined to produce embryonic and extraembryonic tissues, it stimulates trophoectodermal growth. In vitro, the removal of the ICM causes maturation of the trophoblastic cells, inducing them to turn into trophoblastic giant cells that are unable to invade the endometrium. For proper endometrial attachment, the blastocyst should remain attached to the trophoectoderm cells that are adjacent to the inner cell mass. Attachment of trophoblastic cells remote from the ICM has been associated with abnormal placental shape and eccentric insertion of the umbilical cord [81].
Prior to blastocyst attachment, for a successful pregnancy in the window of implantation, the uterine epithelium has to retract its cilia and express pinopodes. If all necessary molecular events occur, the blastocyst is firmly attached to the uterine epithelium around 6–7 days postconception.
The trophoblastic cells in contact with the inner cell mass start to proliferate and invade the uterine epithelium. As they invade, they fuse with each other and form multinucleated giant trophoblastic cells, known as syncytiotrophoblasts. An inner layer of mononucleated trophoblastic cells also develops called cytotrophoblasts. With fusion, the multinucleated syncytiotrophoblastic cells cannot proliferate, so the cytotrophoblastic cells function as a reservoir. Throughout the pregnancy, cytotrophoblastic cells divide and replenish the mature syncytiotrophoblastic cells. In addition to replenishing syncytiotrophoblasts, cytotrophoblasts give rise to various other cell types of the placenta, which are discussed below [82]
The fusion kinetics of cytotrophoblasts changes during pregnancy. In early pregnancy, two mononuclear cytotrophoblasts fuse to become a syncytiotrophoblast. However, later in the pregnancy, cytotrophoblasts fuse with already formed syncytiotrophoblasts [82]. Here we will discuss the process of early embryo development; the process of implantation will be discussed in more detail below.
Around 14 days postconception, cytotrophoblastic cells invade beyond the syncytiotrophoblastic cell layer and come into contact with maternal decidual cells. They form a column of cells with a proliferating core, and as the cells proliferate, more mature cells are passively pushed towards the maternal decidua [83]. More immature cells have α(alpha)6β(beta)4 integrin on their surface, which help bind basal membrane components like collagen IV and laminin. However, as they move further in the column and become closer to maternal decidual cells, they change their expression of surface integrins (integrin α1/β1, α5/β1, or α-v/β3/5), which helps them attach to the maternal extracellular matrix [82, 84].
In addition to adhesion molecules, trophoblasts secrete variety of enzymes that regulate invasion. MMP-2 and MMP-9 degrade collagen IV, which is the main collagen component of the basement membrane, and are therefore regarded as key enzymes in the implantation process, enabling the invasion of the trophoblast cells through the decidua and into the maternal vasculature [85, 86]. Tissue inhibitor of matrix metalloproteinases (TIMP), particularly TIMP-1, TIMP-2, and TIMP-3, were also detected in the trophoblastic cells and decidual tissues. TIMPs are normally inhibitory metalloproteinases and their regulation through trophoblastic and decidual cytokines control MMP activity [87–89]. Other lytic enzymes involved in extracellular matrix degradation are urokinase and tissue-type plasminogen activator (uPA and tPA, respectively). Both uPA and tPA are produced by trophoblasts, and their activity is controlled by plasminogen activator inhibitors (PAI) [88]. Another trophoblast protein, adrenomedullin, decreases PAI levels and subsequently increases plasminogen activators. Additionally, adrenomedullin increases trophoblastic proliferation [90].
Trophoblasts also secrete proangiogenic factors, which stimulate new vessel formation during invasion. Neovascularization is essential for the growth and maintenance of the developing embryo. VEGF, PDGF, and PAF are the main angiogenic factors that have been shown to be secreted by trophoblasts. TGF-β(beta) and TNF-α(alpha), which are present in decidua, further stimulate trophoblastic secretion of these angiogenic factors [91].
Complex molecular interactions take place between the decidua and trophoblasts to regulate trophoblastic invasion. In addition to those factors mentioned above, cytokines like EGF, HB-EGF, IGFBP-1, LIF, IL-1 and hormones like hCG and progesterone have also been shown to regulate trophoblast invasion [88].
A number of other important types of trophoblast cells are involved in implantation—namely, extravillous, endovascular, and endoglandular. Small extravillous trophoblasts invade maternal decidua up to the inner one-third of uterine myometrium and reach the maternal spiral arteries. EVTs replace the spiral arteries’ tunica media, which contains mainly the smooth muscle, and transform the spiral arteries into low resistance vessels that are no longer reactive to maternal vasomotor substances. This transformation aims to allow adequate maternal exchange with the developing fetus, particularly in the second trimester when maternal blood flow increases to the uterus to support the developing fetus. Apart from replacing the smooth muscle, endovascular trophoblasts, a subset of EVTs, replace the intimal layer of the spiral arteries [92]. Disturbances in this remodeling can result in IUGR and preeclampsia. Lastly, endoglandular trophoblasts invade the uterine glands, orient them towards the intervillous space, and replace the uterine epithelial cells (◘ Fig. 4.6) [81].

Fig. 4.6
Trophoblastic invasion of maternal decidua. Small interstitial extravillous trophoblasts invade maternal decidua up to the inner one-third of uterine myometrium and replace the tunica media maternal spiral arteries to create low resistance blood flow. Endovascular trophoblasts replace the intimal layer of the blood vessels while endoglandular trophoblasts invade the uterine glands. (Source: Huppertz B, Berghold VM, Kawaguchi R, Gauster M. Am J Reprod Immunol. 2012 May;67(5):349–57. Used with permission from John Wiley and Sons)
Despite all these early trophoblastic changes, free transfer of maternal blood is only established towards the end of the first trimester. The large number of endovascular trophoblasts plugs the distal segments of the spiral arteries during initial invasion. Rather than maternal blood, the intervillous space ultimately contains glandular secretion products and maternal plasma filtrate, which are responsible for intrauterine nutrition, up until approximately 10 weeks gestation [81]. The reasoning behind the initial spiral artery plugging is believed that it helps keep a low oxygen environment and thus decrease free-radical formation during early embryogenesis.
After 10 weeks, the trophoblastic plugs dissolve and maternal blood contributes to intervillous fluid, which provides the appropriate amount of nutrients and oxygen for the developing fetus. These carefully regulated interactions between the invading trophoblasts and the maternal decidua eventually create a functional placenta, which is the main organ of nutrition, respiration, metabolite excretion, and hormone production in the developing fetus.
4.16 Implantation
Implantation can be divided into three stages: apposition, adhesion, and invasion. Apposition is the initial adhesion of the blastocyst to the endometrial surface. Apposition is unstable and with uterine flushing the blastocyst can be detached from the endometrial surface. Apposition is followed by the adhesion stage, when a stronger connection is established between the embryo and endometrium. Finally, in the invasion stage, trophoblastic cells invade the endometrium.
4.17 Endometrial Receptivity
Successful implantation requires a properly developed blastocyst , a receptive endometrium, and series of molecular interactions. In humans, 75% of the failed pregnancies are considered to be secondary to implantation failure, therefore it is essential to understand the basic molecular interactions involved in the process [93]. Under the influence of estradiol, the endometrium proliferates and reaches a critical thickness to support implantation. After ovulation, in response to progesterone, the endometrium differentiates and becomes receptive to the newly hatched blastocyst.
Implantation occurs around 6 days after ovulation, ranging between 6 and 12 days [94]. The ideal time for implantation is thought to be around day 7 to day 9 after the LH surge and is called the “window of implantation.” This period is characterized by structural and secretory changes in endometrial cells, providing the most favorable conditions for successful blastocyst implantation. The endometrium increases in thickness, becomes more vascularized, and glands become tortuous and increase their secretions rich in cholesterol, fat-soluble vitamins, lipid, and protein. These secretions will serve as an energy source for the embryo, which has no connection to uterine vessels at this point in development. In addition, there is a decrease in uterine fluid content to allow greater contact between the embryo and endometrium. These drastic changes in the uterine environment are mostly the result of progesterone stimulating the differentiation of endometrial cells into decidual cells. Decidual cells contain more intracellular lipids and glycogen deposits than endometrial cells, which cause them to get a polygonal shape as opposed to more rounded endometrial cells.
4.18 Pinopodes
One characteristic feature of receptive endometrium is the presence of pinopodes on the apical surface of endometrial cells. Pinopodes are bleb-like protrusions into the uterine lumen and are found in large numbers between days 19 and 21 in an idealized 28-day menstrual cycle. Although they are expressed throughout the mid- and late secretory phase, they show different morphological features. This suggests that their morphology, rather than their presence, is important for successful implantation [95]. Blastocyst attachment has been shown to occur preferentially on the top of pinopodes, which suggests that receptors necessary for attachment are located on the pinopode surface [96].
Pinopode development has been associated with progesterone [95], HOXA-10, LIF [97], and aVβ3 integrin [98]. HOXA-10, a homeobox gene, is necessary for blastocyst implantation, endometrial stromal cell proliferation, and epithelial cell morphogenesis [95]. Blocking HOXA-10 expression greatly decreases the number of pinopodes.
4.19 Selectins
Selectins are glycoproteins that have a glycosylated extracellular domain, single spanning transmembrane domain, and a cytoplasmic tail. There are three distinct selectins: P selectin, L selectin, and E selectin. Selectins are commonly known for their role in initial leukocyte attachment and subsequent rolling on the endothelial surface. In addition to leukocytes, however, selectins are thought to be responsible for the initial blastocyst -endometrium attachment.
Strong L selectin expression has been shown on the blastocyst surface, whereas on the maternal site, its ligands, namely MECA-79 and HECA-452, are up-regulated during the window of implantation [99]. Although L selectin is found on both luminal and glandular epithelium, expression of L selectin is higher on the luminal epithelium [100]. Initial trophoblast attachment to endometrium is thought to occur with trophoblastic L selectin and endometrial oligosaccharide interactions [101].
4.20 Integrins
Integrins are transmembrane glycoproteins composed of noncovalently linked α(alpha)- and β(beta)-subunits. Each subunit has an extracellular, intracellular, and transmembrane domain. The intracellular domains are linked to the cytoplasmic cytoskeleton and intracellular signaling pathways [96]. They are paired to compose integrin heterodimers; 24 functionally distinct integrins have been identified [102]. Among various other functions, they are mainly involved in cell-to-cell and cell-to-extracellular matrix interactions. Among the many different types of integrins that are expressed constitutively in the endometrium, α1β1, α4β1, αvβ3 are co-expressed between days 20 and 24 in the menstrual cycle. β(beta)3 integrin deserves special attention among other subunits, because its expression starts at cycle day 19 and increases thereafter [103]. Moreover, it is mainly expressed on the endometrial luminal surface, which suggests that αvβ3 integrin, and its endometrial ligand osteopontin, might serve as a receptor for embryonic attachment [103, 104]. Various studies showed that the αvβ3 integrin is regulated in both a hormonal and paracrine manner. For example, estrogens down-regulate integrin expression, but increasing levels of progesterone in the luteal phase counteract the estrogen effect. Rather than a direct effect, progesterone increases epidermal growth factor and heparin binding growth factor in the uterine stroma, which results in increased αvβ3 levels [103]. The embryo is also actively involved in the β3 subunit regulation, probably with the embryonic IL-1 system [96].
Additionally, HOXA-10 increases the expression of the β3 subunit in endometrial cells [105]. This subunit is the rate-limiting step in αvβ3 integrin production. Considering the important role of αvβ3 integrin in the implantation process, it’s not surprising that it is used as a clinical marker of endometrial receptivity [96, 106].
4.21 Mucins
Mucins are heavily glycosylated proteins. Carbohydrates constitute 50–90% of their molecular weight. To date, 18 mammalian mucin genes have been identified [107]. Mainly mucin-1 (MUC1) and to lesser extent mucin-6 (MUC6) are expressed in the human endometrium. They are found on the luminal surface of the epithelial cells in the reproductive tract. Their proposed physiological role in the reproductive tract is to trap bacteria and viruses and expel them. They are resistant to digestive enzymes. Their extracellular portion can be cleaved, and those cleaved molecules can join via sulfide bonds to create a mucin gel. Altogether, mucins produce a formidable barrier in microbial defense. Estrogens increase mucin production. Progesterone has no independent effect on mucin production; yet by counteracting the effects of estrogens, the net effect of progesterone is to decrease mucin levels. Cytokines, particularly TNF-α, have also been shown to be involved in mucin regulation.
Although they provide an important barrier in microbial defense, mucins also constitute a barrier against blastocyst implantation. Mucins extend their projections well beyond endometrial surface receptors, thereby hindering blastocyst access to them [108]. At the site of implantation, mucins’ extracellular domain needs to be cleaved. The sheddase family of enzymes, particularly TACE/ADAM17 and MT1-MMP, has been suggested to play a role in this cleavage process [109]. The blastocyst, through the action of secreted cytokines, up-regulates sheddases that cleave mucins in endometrium [108].
Interestingly, during the implantation period, mucin production is increased [110]. It seems to be a paradoxical phenomenon; however, two possible explanations have been suggested. First, after sexual intercourse, the ejaculate may introduce microbial pathogens into the endometrium and the increased mucin levels may act as an additional protective barrier . Second, as the blastocyst is actively involved in sheddase induction, it has to be competent to do so. Mucins may be a protective mechanism against the attachment of unhealthy embryos that would otherwise have resulted in pregnancy failure [110]. Consistent with this view, women with recurrent pregnancy failure have decreased mucin levels compared to a fertile control group [111].
To summarize, mucins prevent embryo attachment and need to be cleaved at the site of embryonic attachment. This process involves a series of interactions that requires a healthy embryo as well as a functional endometrium.
4.22 Cytokines
Cytokines are soluble proteins that have a variety of functions in inflammation, the menstrual cycle, ovulation, and implantation. A disturbance in the normal expression or action of several cytokines results in implantation failure and abnormal placental development in humans. Of known importance are members of the gp130 family, such as LIF, IL-1, IL-11, and IL-15 system [112].
4.23 Leukemia Inhibitory Factor
A member of the gp130 cytokines, LIF acts through its surface receptor complex, LIF receptor (LIFR), and the gp130 receptor chain. Binding of LIF to LIFR results in heterodimerization with gp130 and subsequent activation of downstream signaling pathways that include the JAK/STAT, MAP kinase, and PI3 kinase pathways [96]. Other members of the gp130 cytokine family, including oncostatin M, ciliary neurotrophic factor, cardiotrophin-1, IL-6, and IL-11, can also bind to the LIFR [112].
LIF was the first cytokine shown to be critical for implantation in mice [113]. Wild-type mice embryos failed to implant in the endometrium of homozygous LIF mutant female mice, and the implantation failure was reversed after LIF supplementation .
LIF mRNA is expressed between menstrual cycle days 18 and 28 in fertile women , and it is expressed by both glandular and luminal epithelium [114]. Among many LIF regulators, progesterone is probably responsible for endometrial LIF induction. When treated with a selective progesterone receptor modulator, mifepristone, decreased levels of LIF are observed in endometrium [115]. In addition to progesterone, IL-1α, TNF, PDGF, TGF-β1, and HB-EGF stimulate LIF expression in cultured endometrial stromal cells. The embryo secretes hCG, IGF-1, and IGF-2 that also increases LIF levels [116].
LIF protein expression is maximal in uterine flushings in the midlate secretory phase of the menstrual cycle at the time of expected implantation. Considering the ease of performing uterine flushings, LIF has been suggested as a marker of uterine receptivity [117, 118]. In women with recurrent implantation failure, LIF levels are lower than in controls, emphasizing the importance of LIF in successful implantation [118, 119]. rhLIF has also been suggested to improve endometrial receptivity in recurrent implantation failure patients; however, the efficacy has not been demonstrated in clinical trials [120].
4.24 Interleukins
IL-1 is one of the key regulatory mediators of the inflammatory response. IL-1α, IL-1β, and the IL-1 receptor antagonist are members of the IL-1 cytokine family. Stromal cells, glandular cells, and macrophages are the reservoir of IL-1 in the endometrium. In vitro, treating endometrial cells with IL-1 increases integrin β3 expression in the epithelial cells [121]. IL-1α knockout mice are, however, fertile, suggesting redundancy in the effects these interleukins have in implantation. IL-1 receptor antagonist expression is decreased during the implantation window. It is possible that down-regulation of IL-1 antagonist works synergistically with IL-1 to affect implantation [122]. Exogenous IL-1 receptor antagonist treatment during implantation can block blastocyst implantation [121]. Overall, the IL-1 system is clearly involved in implantation; however, its exact role in implantation remains unclear.
IL-6 is involved in many immune interactions, and it has been also suggested to play a role in implantation. Endometrial IL-6 mRNA expression increases during the mid- to late secretory phase and decreases in the late secretory phase. Strong immunoreactivity has been observed in uterine glandular and luminal epithelium during the window of implantation [123]. While controversial, IL-6-deficient mice appear to have reduced fertility and decreased implantation rate [124]. The IL-6 receptor is found on the surface of the blastocyst , and IL-6 is probably involved in paracrine/autocrine interactions in the window of implantation . Decreased levels of mid-secretory IL-6 mRNA are found in patients with recurrent spontaneous abortions, also supporting this hypothesis [125].
Another cytokine that has gained attention is IL-11. IL-11 has anti-inflammatory activities, and it is expressed in endometrial glandular and luminal epithelium. Estrogen, progesterone, and local factors increase IL-11 levels. IL-11 advances progesterone-induced decidualization of human endometrial stromal cells. IL-11 and its receptor IL-11R were immunolocalized to decidualized stromal cells in the mid-late secretory phase epithelium. They were also shown on the trophoblastic cells, suggesting a role in normal placentation [112]. Additionally, inadequate IL-11 signaling was found to result in dysregulation in trophoblastic invasion [126].
4.25 Prostaglandins
Prostaglandins (PGs) are lipid mediators of inflammation, and they have a variety of functions in inflammation, menstrual cycle regulation, ovulation, embryo attachment, trophoblastic invasion, and labor. Prostaglandins, leukotrienes, and thromboxanes are members of the eicosanoid family. They are produced from membrane lipids by phospholipase A2 (PLA2) and cyclooxygenase (COX) enzymes. To date, three isoforms of COX enzymes have been discovered: COX-1, COX-2, and COX-3. COX-1 is constitutive and expressed under normal physiological functions, whereas COX-2 is involved mainly in inflammatory responses. COX-3 is expressed in the human brain and is responsible for fever and response to pain.
Murine studies have shown the importance of prostaglandins (PGs) in implantation. Lack of either PLA2 or COX2 in mice leads to defective PG synthesis; PLA2 knockout mice show pregnancy failure [127]. COX expression is maximal in the menstrual and proliferative phases . Among many regulators, IL-1 deserves further consideration. IL-1 increases COX enzymes and PG production, resulting in increased endometrial integrin levels that are essential for blastocyst implantation [96].
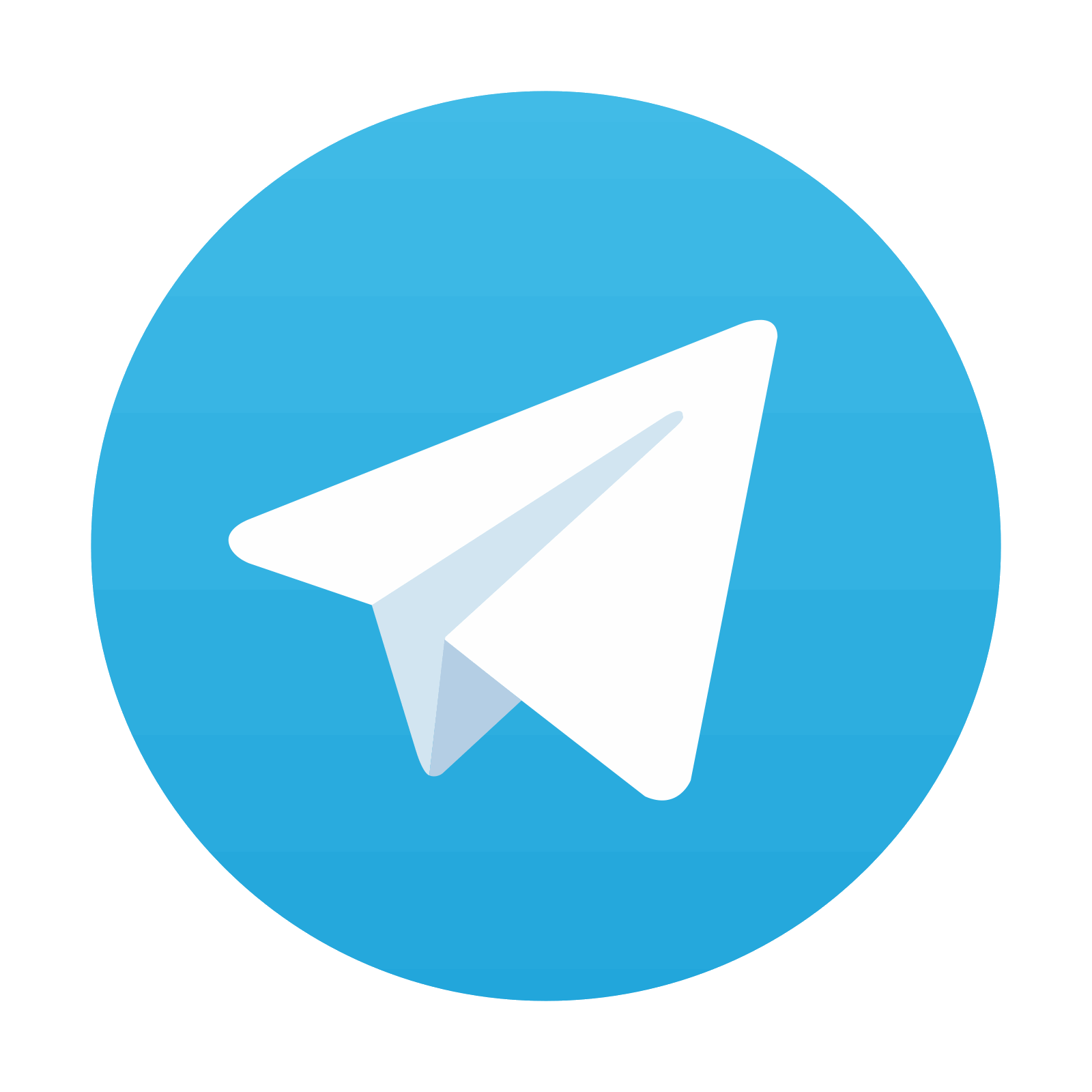
Stay updated, free articles. Join our Telegram channel
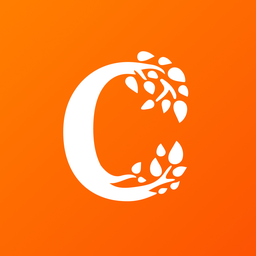
Full access? Get Clinical Tree
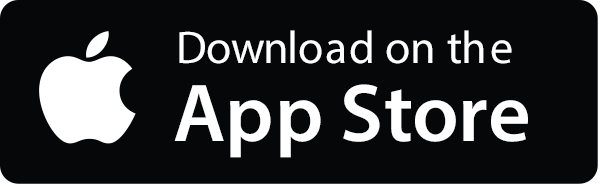
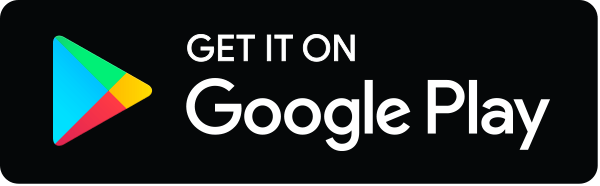