Chapter 15: Clinical applications of near-infrared spectroscopy in neonates
Petra Lemmers, Suresh Victor, Michael Weindling, Laura Dix, Frank van Bel, Gunnar Naulaers
Key points
- • The status of cerebral oxygenation is not always represented appropriately by systemic arterial oxygenation, especially when there are changes in cerebral blood flow or cardiac output. Oxygenation monitoring of the brain by near-infrared spectroscopy (NIRS) is therefore an important additive measure in neonatal intensive care.
- • Monitoring cerebral oxygenation by NIRS, in addition to arterial saturation monitoring by pulse oximetry, blood pressure, and brain function by aEEG, can help to prevent brain damage as well as prevent unnecessary treatment of the neonate.
- • Cerebral oxygenation can be stabilized in the neonate using a dedicated treatment guideline in combination with cerebral oxygenation monitoring by NIRS.
Introduction
Survival of the extremely preterm infant has greatly improved over the past decades. However, perinatal brain damage with adverse neurodevelopmental outcome continues to affect a considerable number of these infants.1–12 Although the etiology of brain damage is multi-factorial and partly unknown (see Chapter 7), hypoxia, hyperoxia, specific and non-specific inflammation, and hemodynamic instability during the first days of postnatal life play an important role.13–19 It is clear that further advances in survival and improvements in neurodevelopmental outcome can only be achieved if we learn more about the underlying pathophysiology so that more effective treatment modalities can be established. The first step in this direction is to develop the capability to continuously monitor clinically relevant hemodynamic variables and, if possible, treat the underlying condition at an early stage. Continuous monitoring of physiological parameters such as heart rate, blood pressure, arterial oxygen saturation (SaO2), temperature, and, with increasing frequency, electrical activity of the brain using amplitude-integrated EEG (aEEG) have been integrated into the monitoring practices of neonatal intensive care units (NICUs). aEEG has been introduced into neonatal intensive care as a novel monitoring technique to continuously assess cerebral electric activity. Both the aEEG background patterns and the analysis of the raw EEG signal have been used for the evaluation of neurological function. The fewer channels compared to the classic full EEG improves its applicability, and the use of aEEG has increased.20,21 Other, novel techniques used to continuously monitor additional hemodynamic parameters, such as cardiac output, are discussed in Chapters 9–11 in detail.
Intermittent tools to assess cerebral health, such as cranial ultrasound, Doppler flow velocity measurements, and (advanced) magnetic resonance imaging (MRI), have also been integrated into the care of the sick neonate (see Chapters 11–13 for details). These techniques, however, do not provide continuous information on the perfusion and oxygenation of the neonatal brain.
Therefore we need a reliable and practical clinical tool that monitors oxygenation of the neonatal brain noninvasively and continuously so that conditions potentially leading to brain injury can be recognized in a timely manner. An increasingly clinically used method is monitoring cerebral oxygenation by NIRS.22–26
Principles of near-infrared spectroscopy
Near-infrared (NIR) spectroscopy technology utilizes light in the near-infrared range (700–1000 nm). NIR spectroscopy instrumentation consists of fiber optic bundles or optodes placed either on opposite sides of the tissue being interrogated (usually a limb or the head of a baby) to measure transmitted light or close together to measure reflected light. NIR-light (laser or, more frequently, LED light) enters through one optode and a fraction of the photons are captured by a second optode and conveyed to a measuring device.
Jöbsis first introduced the use of NIR spectrophotometers for human tissue in 1977.27 Human tissues contain a variety of substances whose absorption spectra at NIR wavelengths are well defined. They are present in sufficient quantities to contribute significant attenuation to measurements of transmitted light. The concentration of some absorbers such as water, melanin, and bilirubin remains virtually constant with time. However, the concentrations of some absorbing compounds, such as oxygenated hemoglobin (HbO2) and deoxyhemoglobin (HbR), vary with tissue oxygenation. Therefore changes in light absorption can be related to changes in the concentrations of these compounds.
The absorption properties of hemoglobin alter when it changes from its oxygenated to its deoxygenated form. In the NIR region of the spectrum the absorption of the hemoglobin chromophores (HbR and HbO2) decreases significantly compared to that observed in the visible region. The major part of the NIR spectroscopy signal is derived from hemoglobin, but other hemoglobin compounds, such as carboxyhemoglobin, also absorb light in the NIR region. However, the combined error due to ignoring these compounds in the measurement of the total hemoglobin signal is probably less than 1% in normal blood.
Near-infrared spectrophotometers
Three different methods of using NIR light for monitoring tissue oxygenation are currently used: continuous wave,28–30 time-of-flight (also known as time-domain or time-resolved),31 and the frequency domain methods.30 For an extensive overview of the methods, we refer the reader to Wolf et al.32 The continuous wave method has a very fast response but registers relative change only, and it is therefore not possible to make absolute measurements using this technique. The time-of-flight method needs extensive data processing but provides more accurate measurements. It enables one to explore different information provided by the measured signals and has the potential to become a valuable tool in research and clinical environments. The third approach, which uses frequency domain or phase modulation technology, has a lower resolution than that of the time-of-flight method but has the potential to provide estimates of oxygen delivery sufficiently quickly for clinical purposes. Thus frequency domain or phase modulation technology is potentially the best candidate in the NICU and for bedside usage. Nevertheless, the continuous wave method devices have been widely used for research studies.25,33–39
Continuous wave spectroscopy
In continuous wave spectroscopy changes in tissue chromophore concentrations from the baseline value can be obtained from the modified Beer-Lambert law28. However, the application of the Beer-Lambert law in its original form has limitations. Its linearity is limited by deviation in the absorption coefficient at high concentrations, scattering of light due to particulate matter in the sample, and ambient light.
Thus for light passing through a highly scattering medium, the Beer-Lambert law has been modified to include an additive term, K, due to scattering losses, and a multiplier to account for the increased optical pathlength due to scattering.40–42
The modified Beer-Lambert law is expressed as A = P × L × E × C + K, where A is absorbance, P is the pathlength factor, L is the path length, E is the extinction coefficient, C is the concentration of the compound, and K is a constant. The differential pathlength factor describes the actual distance traveled by light. As it is dependent on the amount of scattering in the medium, its measurement is not straightforward. Examples of instruments using continuous wave technology are the NIRO 500 and NIRO 100, made by Hamamatsu Photonic, Hamamatsu, Japan.
Spatially resolved spectroscopy
In spatially resolved spectroscopy multiple optodes, operating simultaneously, are placed around the head. This allows for a pathlength correction, assuming that tissue being interrogated is homogeneous, the so-called spatially resolved spectroscopy.43,44 Spatially resolved spectroscopy measures hemoglobin oxygen saturation. The NIRO 300 (Hamamatsu Photonics, Hamamatsu City, Japan) and the INVOS 5100 (Somanetics, Troy, MI, United States) both measure cerebral hemoglobin oxygen saturation but use different terminology: tissue oxygenation index (TOI) from the NIRO 300 and regional cerebral oxygen saturation (rSO2) from the INVOS 5100. This technique gives absolute values. A light detector measures tissue oxygenation index with three sensors at different distances from the light source. Tissue oxygenation index (TOI) is calculated according to the diffusion equation as follows: TOI (%) = KHbO2/(KHbO2+KHbR), where K is the constant scattering contribution and HbO2 and HbR are the oxygenated and deoxygenated hemoglobin, respectively. A similar concept is used in calculating regional oxygen saturation (rSO2). There was good agreement for sensor-exchange experiments (removing and reapplying the sensor at same position); simultaneous left-to-right forehead measurements and sensors at different positions revealed acceptable and insignificant differences.24,45 Quaresima and colleagues concluded that TOI reflected mainly the saturation of the intracranial venous compartment of circulation.46 Variation in the results of studies were likely due to assumptions made about the distribution of cerebral blood between arterial and venous compartments.47,48 Several other commercial instruments are on the market now, most of them using the same physical principles, although using different distances, algorithms, and probes. There are now several commercial monitors in use in the NICU.49 Although different terms are used (TOI, StO2, rSO2), we will use rSO2 further in the text when we talk about cerebral oxygenation.
Time-of-flight instruments
This time-resolved technique consists of emitting a very short laser pulse into an absorbing tissue and recording the temporal response (time-of-flight) of the photons at some distance from the laser source.31 There have only been a few reports on the use of time-of-flight instruments in neonates.44,50,51
Frequency domain instruments
The frequency domain method is based on the modulation of a laser light at given frequencies.30 Frequency domain instruments determine the absorption coefficient and reduce the scattering coefficient of the tissue. Problems include noise and leakage associated with the high-frequency signal, but the devices are very compact and appropriate for bedside/incubator use. Frequency domain NIRS allows the absolute quantification of cerebral hemoglobin oxygen saturation and cerebral blood volume.52–54 Using concurrent frequency domain measures of cerebral hemoglobin oxygen saturation and diffuse correlation spectroscopy measures of CBFi, cerebral oxygen consumption can be calculated.55–57
Near-infrared spectroscopy in clinical care
The introduction of spatially resolved spectroscopy made it possible to use a new approach to monitoring cerebral oxygenation in the clinical setting. It measures the status of cerebral oxygenation by using regional cerebral oxygen saturation (rScO2). Despite the different approaches, the measures of cerebral oxygenation reflect mixed tissue oxygen saturation by assuming that the contribution to the perfusion of the tissue interrogated is 25%, 5%, and 70% by the arteries, capillaries, and veins, respectively. The value is provided as an absolute number that can be measured continuously and over prolonged periods of time.27
For most of the instruments, a good correlation with jugular venous O2 saturation has been documented.58,59 The values are not identical, though primarily because TOI and rScO2 reflect the changes in oxygenation in the arterial, capillary, and venous compartments. A comparison between the different monitoring techniques in adults during changes in oxygenation and changes in partial pressure of arterial CO2 (PaCO2) also yielded a good correlation between the TOI and rScO2.47,48 In addition, when comparing the left and right sides of the brain, the Bland-Altman limits of agreement for rScO2 were −8.5 to + 9.5%, with even smaller limits during stable SaO2 values between 85 and 97%.45 However, due to the limitations of the technology, it is obvious that these measurements are better used for trend measurements rather than precise tissue oxygenation values.
Clinical monitoring of cerebral oxygenation by NIRS has already become routine in pediatric and adult intensive care and during cardiac surgical procedures in all age groups.47,60–62 However, although information concerning brain tissue oxygenation may be important when considering the type and timing of an intervention and when assessing its impact on outcome, the use of NIRS has not yet been universally implemented in the daily care of neonates in the NICU, but the use in NICU’s in Europe and the United States is increasing. Although the accumulating evidence supporting the use of NIRS in clinical practice is encouraging, more research is necessary to further consolidate its clinical importance.
In addition to rScO2, cerebral fractional tissue oxygen extraction (cFTOE) is another important NIRS parameter. Cerebral fractional tissue oxygen extraction is derived from rScO2 and SaO2 based on the formula: SaO2 − rScO2/SaO2. Thus cFTOE is a surrogate indicator of the actual cerebral fractional oxygen extraction, which can be measured with the validated jugular venous occlusion technique.59,63 Naulaers et al. reported a positive correlation between NIRS-calculated cFTOE and actual fractional oxygen extraction of the brain in a newborn piglet model.64 Because cFTOE is a ratio of two variables, an increase might either indicate reduced oxygen delivery to the brain with constant oxygen consumption or increased cerebral oxygen consumption not satisfied by oxygen delivery. The opposite is true in the case of a decrease in the cFTOE, reflecting either a decrease in oxygen extraction because of decreased oxygen utilization or an increase in oxygen delivery to the brain while cerebral oxygen consumption has remained unchanged. Obviously, either parameter might change at the same time, although relatively rapid changes in cerebral oxygen utilization are less common. Although NIRS-derived cFTOE is a less accurate parameter compared to fractional oxygen extraction determined by the jugular occlusion technique, the advantage of NIRS-derived cFTOE is that cerebral oxygen extraction can be continuously assessed.38,65
Feasibility of NIRS-monitored cerebral oxygenation and extraction in neonatal clinical care
Cerebral oxygenation and extraction can be monitored by NIRS-derived rScO2 and cFTOE, respectively. In order to assess the utility of NIRS-monitored rScO2 in clinical practice, it is essential to also obtain data on the signal-to-noise ratio and the inter- and intra-patient variability. When compared to pulse oximetry monitored SaO2, it is a reliable and accepted trend monitor for systemic arterial oxygenation, but the signal-to-noise ratio is larger for NIRS-measured rScO2.24 However, when averaging the signal over a longer period, for example, over 30–60 seconds, a reliable NIRS signal can be obtained with an acceptable signal-to-noise ratio.24 With respect to intra-patient variability, differences of up to 7% or more have been reported when performed with repeated placement of the NIRS sensor.63 The limits of agreement after sensor replacement are in the −17% to +17% range.24,66,67 These values are more than double that of the limits of agreement for SaO2.63 On the other hand, Menke et al reported a good reproducibility of NIRS-measured rScO2 with an inter-measurement variance only slightly higher than the physiological baseline variation.68 When comparing values during simultaneous monitoring of the left and right frontoparietal regions of the brain, limits of agreement of 7–9% have been reported.45 Moreover, it appears that the experience of the investigator also plays an important role in the quality of the information obtained.
Reference values of rScO2 or TOI during normal arterial oxygen saturations have been reported in several studies including preterm neonates. Of note is the fact that not all studies incorporated postnatal age or the clinical status of the infant when reporting their findings. Mean values (± SD) of rScO2 or TOI ranged between 61 and 75% (±7 to ±12%). These values are comparable to those obtained in adults (Table 15.1).45,48,66,67,69–76
The inter-patient variance of TOI/rScO2 in these studies was larger than for pulse oximetry–measured oxygen saturation. Reference values for preterm infants are within the same range as the reference values of adults, infants, and term neonates. See text for details.
Over the past decade or so, more and more NIRS devices with neonatal or pediatric sensors and algorithms have become available. Before interpreting the absolute values, though, attention must be paid to the differences between the adult and new neonatal sensors. Indeed, studies have shown that the newer, smaller neonatal sensors may measure at up to 10–15% higher values compared to the previously used adult sensors in neonates.74,77 Therefore reference values for the neonatal or pediatric sensors in preterm and term neonates are necessary before their implementation in clinical practice. Indeed, a large study by Alderliesten et al. on 999 preterm infants has recently provided reference values for rScO2 and cFTOE for different types of sensors.75,78 Graphs of reference value curves allow for bedside interpretation of NIRS-monitored cerebral oxygenation (Table 15.1). These reference values can then be used for comparison with values obtained during conditions that may affect cerebral oxygenation (see below).
Another important use of cerebral oxygenation measurement is to avoid cerebral hypoxia. Therefore the threshold for cerebral hypoxia needs to be determined.
Several animal studies in newborn piglets and one human study in neonates with hypoplastic left heart syndrome who underwent open heart surgery have reported that rScO2 values lower than 35–45% for more than 30–90 minutes are associated with functional (mitochondrial dysfunction or energy failure) and/or histological damage, especially in the hippocampus (a brain region very vulnerable to hypoxia in the perinatal period).79–81 Moreover, a number of studies, mostly performed in adult cardiac intensive care units, have reported that a 20% decrease from the baseline or an absolute rScO2 value of <50% before intervention is associated with hypoxic-ischemic brain lesions.82 Thus these findings suggest that rScO2 values below 45–50% for a prolonged period of time should be avoided if possible. A large international randomized controlled clinical trial has investigated if it is possible to detect and prevent cerebral oxygenation outside the assumed normal limits of 55–85% with NIRS monitoring (measured with the adult sensor) to prevent neurological injury and improve neurodevelopmental outcome.83 The SafeBoosC II trial (safeguarding the brains of our smallest children) randomized infants to either visible or shielded NIRS recording of cerebral oxygenation and then compared the burden of hypoxia and hyperoxia between the two groups.84 Infants with visible NIRS tracings spent a shorter time outside the normal range, mainly due to a reduction in the hypoxic burden due to clinical interventions. These findings indicate that unfavorable cerebral oxygen saturations can be detected by NIRS and prevented by timely interventions.84 Because different monitors have different values, a liquid phantom was used to compare the absolute values and to define hypoxia thresholds for the different monitors.49 In this way the hypoxia threshold for every monitor is defined (Figure 15.1).
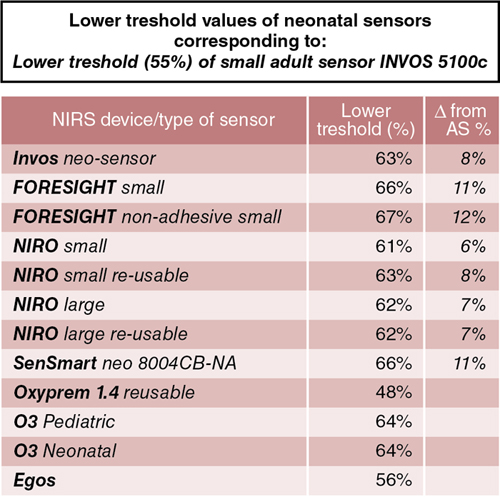
Similar to the bedside detection and prevention of prolonged cerebral hypoxia, continuous monitoring of rScO2 can also contribute to the prevention of prolonged cerebral hyperoxia, especially in the extremely preterm infant who is particularly prone to oxygen toxicity. The importance of avoiding hyperoxia has been increasingly recognized, as an association between normal oxygen saturations and improved long-term neurodevelopmental outcome in extremely preterm infants has been documented.19,85,86 With these considerations and the presented data in mind, NIRS-monitored rScO2 and NIRS-derived cFTOE are likely to play an important role in monitoring and improving cerebral oxygenation in sick neonates in the future.
Clinical applications
Application of the sensor and its pitfalls
The most important issue regarding the clinical application of noninvasive monitoring of cerebral oxygenation by NIRS is the ability to perform reliable, long-term monitoring in the most immature and unstable neonate without disturbing the infant. A critical part of initiating the process is the application of the sensor to the head. With appropriate placement, the sensor will allow reliable monitoring of the rScO2 for a number of days without damaging the vulnerable skin, particularly of the very preterm infant. In addition, the sensor in place should not limit access to performing ultrasound studies of the brain, placement of electrodes for aEEG monitoring, and attachment of CPAP devices. In our experience, application of the NIRS sensor with a soft dark elastic bandage to the frontoparietal region of the head provides protection from ambient light and doesn’t irritate or damage the skin of even the smallest infants, while allowing reliable monitoring of rScO2 for extended periods of time. Figure 15.2 shows an example of the application of the NIRS sensor used in all of our clinical studies.22 Alternatively, application of sensors using the original adhesive tape on the skin is possible and this method is advocated by the manufacturers for most commercially available sensors (Figure 15.2).
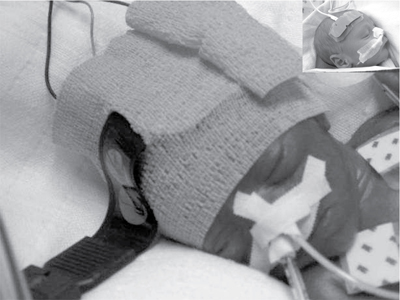
Introduction of the system with structured theoretical courses and practical training for nurses and medical staff is a very important prerequisite for the successful use of this monitoring method in clinical practice. In addition, staff education about the potential benefits and risks of using NIRS is of great importance. In gaining experience nursing staff in the authors’ institutions have been able to recognize inappropriate transducer placement, improper transducer fixation, or insufficient transducer shielding. In our experience this resulted in extended periods of uninterrupted and reliable rScO2 monitoring, even in the smallest infants, and is comparable to SaO2 monitoring. When interpreting the values in daily clinical practice, one has to be aware of several pitfalls. We have already discussed the importance of proper sensor application to prevent movement artifacts and the effect of ambient light. Yet, despite these precautionary measures, phototherapy light will sometimes cause disturbances in rScO2 monitoring. Accordingly, covering the sensor with an additional dark sheet during periods of phototherapy is recommended to ensure more reliable signal acquisition. Other factors such as dislocation of the NIRS sensor, presence of hair, hematoma or edema, and/or other materials such as the plasters of the aEEG electrodes on the head can also cause disturbances of the NIRS signal. Interestingly, the influence of the curvature of the skull and head circumference seems negligible.75
Relation to other monitoring devices
NIRS-monitored changes in brain oxygenation, represented by rScO2 (or TOI) and cFTOE, generate important continuous information in addition to data from other monitoring devices such as pulse oximetry–monitored SaO2, indwelling blood pressure monitoring, and heart rate and electrical brain activity monitors. Monitoring SaO2 is necessary to calculate cFTOE as described earlier. The relationship between blood pressure and rScO2 may provide information about the presence or lack of cerebral blood flow autoregulation (see below and Chapter 2).87–90 As for the use of aEEG in combination with NIRS, our group has reported that persistent and unusually high rScO2 values in term infants with severe perinatal asphyxia are probably due to profound vasodilation with or without vasoparalysis of the cerebral vascular bed. A decreased utilization of oxygen is also strongly associated with an abnormal aEEG pattern after the first postnatal day and adverse neurodevelopmental outcome at 2 years of age.91,92 These findings indicate that monitoring cerebral oxygenation and oxygen extraction with NIRS along with other parameters reveals conditions at an early stage that might be associated with poor long-term outcomes.84 The combination of the different parameters monitored can also be used in pharmacodynamic research. Medications given to neonates often have effects on both cerebral metabolism and hemodynamics, so using the combination of NIRS, aEEG, and hemodynamic parameters like blood pressure and heart rate will provide additional valuable information. An example is the use of propofol, which has direct effects on both blood pressure and cerebral metabolism.93–95 Further research in neurovascular coupling in preterm infants is ongoing.96,97 Finally, efforts to develop a comprehensive, real-time cardiorespiratory and neurocritical care monitoring system are described in Chapter 14.
Clinical conditions associated with low rScO2
Clinical conditions and treatment guidelines were well described in the SafeboosC II and III study.98
Low rScO2 can be caused by low oxygen levels in the blood (hypoxic hypoxia), anemia (anemic hypoxia), or low cerebral blood flow (ischemic hypoxia).
Hypoxic hypoxia is the most frequent cause, as was shown in the SafeboosC II study.99 This is evident as a decrease in oxygen saturation immediately causes a decrease in cerebral oxygenation.72 First-line approach to treatment should focus on optimizing pulmonary blood flow and lung recruitment using FiO2 or altering mechanical ventilation strategy.
Anemic hypoxia is a less frequent cause of cerebral hypoxia. Anemia may have an even more profound effect on cerebral oxygen delivery in neonates with hypotension receiving mechanical ventilation with high mean airway pressures. Studies have shown an improvement in cerebral oxygenation following red blood cell transfusion, with the strongest effect in infants with the lowest pre-transfusion cerebral oxygen saturation.100 Van Hoften et al. have documented normalization of rScO2 following packed red blood cell transfusions in 33 preterm infants and concluded that cerebral oxygenation in these infants may be at risk of hemoglobin (Hb) concentrations of <6 mmol/L (9.7 g/dL).101 As discussed earlier, increased cFTOE (>0.4) is indicative of an imbalance between oxygen supply and demand, which may also improve with red blood cell transfusions.102 This suggests a potential role for NIRS monitoring to identify infants with high cFTOE (and low rScO2) who might benefit from red blood cell transfusions.103,104
Ischemic hypoxia is the most important reason to use NIRS as a diagnostic tool. A decrease in cerebral blood flow cannot be detected by the arterial saturation monitor. In this way a decrease in cerebral oxygenation with a normal arterial oxygen saturation, resulting in an increased FTOE, can be an important sign of low cerebral blood flow. There are several different but important causes of low cerebral blood flow in neonates.
As alluded to earlier, PaCO2 is another important parameter and the most powerful one that influences brain perfusion (Chapter 2). Indeed, changes in PaCO2 cause more robust changes in cerebral blood flow than changes in blood pressure outside the autoregulatory range (Chapter 2). Hypocapnia directly decreases cerebral blood flow by inducing vasoconstriction, thus reducing rScO2 and increasing cFTOE, whereas hypercapnia induces vasodilatation, with increased rScO2 and reduced cFTOE values. Accordingly, even small changes in PaCO2 within the normal range may affect the neonatal brain.105 Therefore monitoring rScO2 and cFTOE together can be used as a noninvasive approach to identify changes in PaCO2, provided that cerebral metabolic rate and the proportion of arterial and venous blood flow in the brain remain constant and hemoglobin concentration and SaO2 don’t change during the period of interrogation.106 Figure 15.3A shows an example of a preterm infant with low PaCO2 and rScO2 values. Only when PaCO2 increased to >30 mmHg did rScO2 recover.
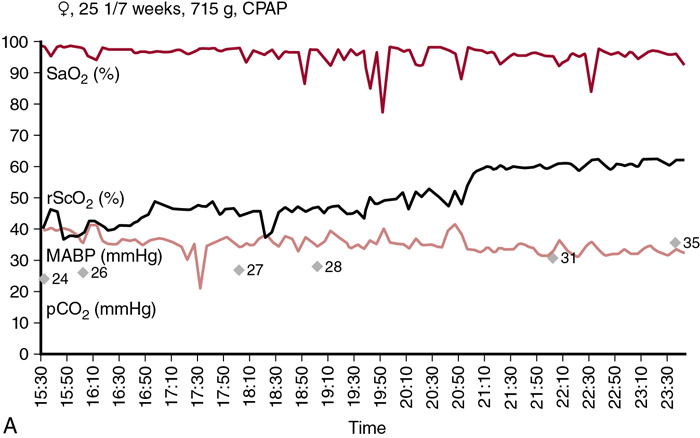
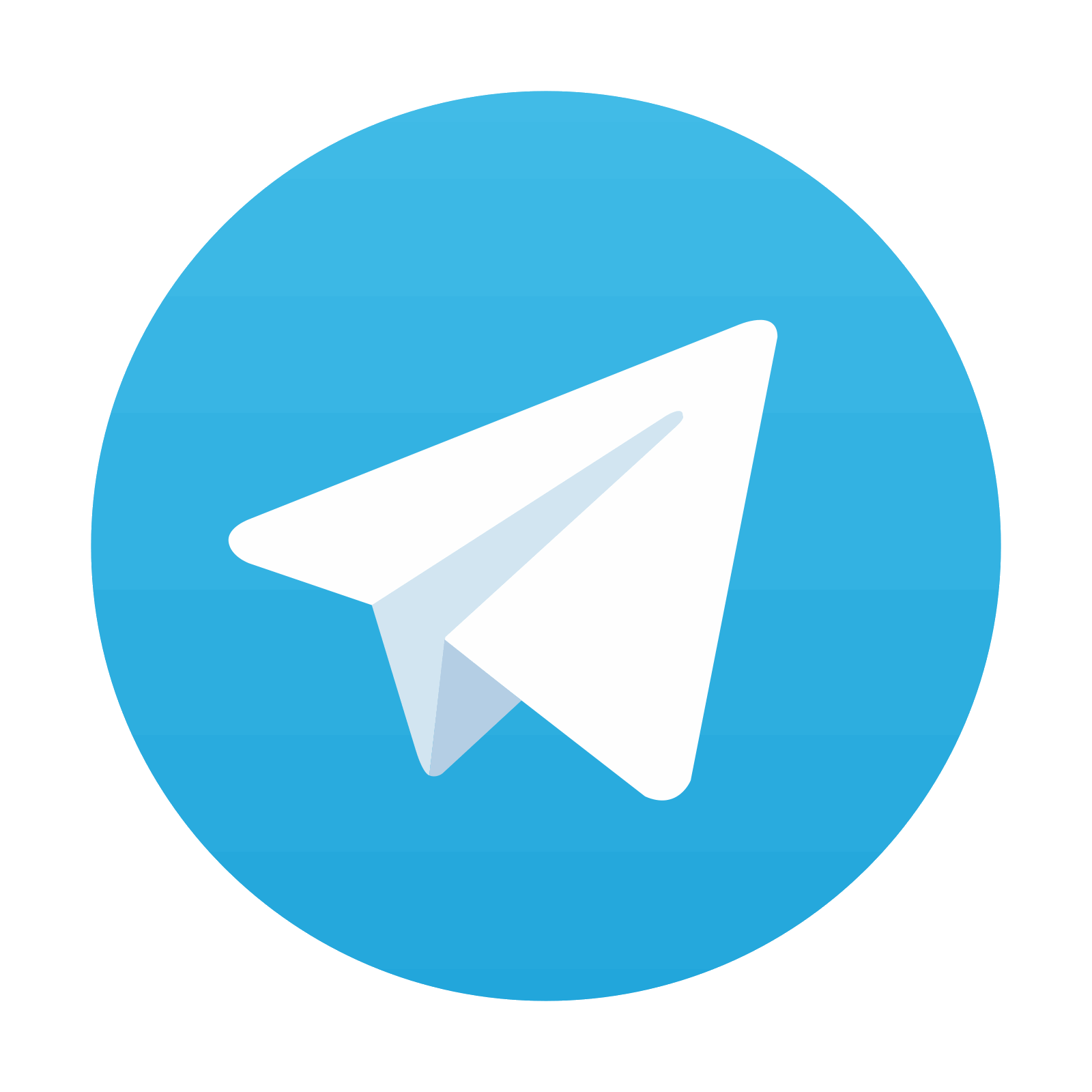
Stay updated, free articles. Join our Telegram channel
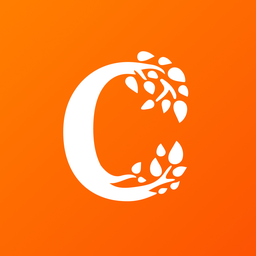
Full access? Get Clinical Tree
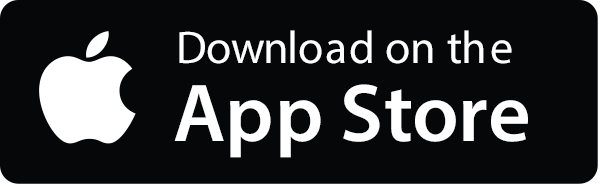
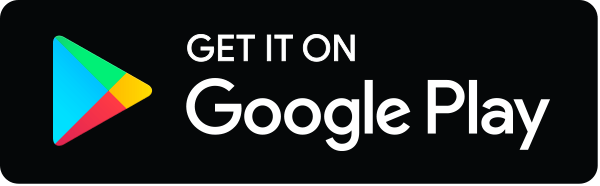