Fig. 23.1
Bone formation (+) and resorption (−) during growth. (From Baron [12]. This material is reproduced with permission of John Wiley & Sons, Inc.)
Changes in Cortical and Trabecular Bone with Growth
Cortical and trabecular bone do not respond in the same way to diseases, medications, or mechanical loading, and should be considered two functional entities. Trabecular volumetric bone mineral density (BMD), as measured by three-dimensional quantitative computed tomography (QCT), does not increase before puberty [13, 14]. During puberty, trabecular BMD increases significantly in healthy children due to increases in trabecular thickness. The increases in BMD is comparable in girls and boys [15], but is significantly greater in black adolescents than in white adolescents [16].
Sex differences in cortical dimensions are established during puberty (Fig. 23.2) [17]: cortical width increases by periosteal bone formation in boys, and by less periosteal bone formation but more endocortical apposition in girls. Androgens stimulate periosteal apposition while estrogens inhibit periosteal apposition and stimulate endosteal apposition. These sex differences have important implications for bone strength; the greater R p in males results in greater bone strength. The long bones are tubular structures that are loaded mainly in bending. The resistance of long bones to bending (i.e., bone strength) is represented by the cross-sectional moment of inertia (CSMI) = π/4 (R p4−R e4); R p and R e indicate the periosteal and endosteal radius, respectively [18]. These power relationships indicate that small increases in R p result in marked increases in bone bending strength.

Fig. 23.2
Sex specific increases in cortical bone dimensions during growth and maturation. (Adapted from Seeman [17] with permission from Elsevier)
Because the patterns of modeling on the periosteal and endocortical envelopes during growth produce changes in cortical geometry that impact life-long fracture risk [19, 20], the long-term effects of chronic childhood diseases, such as IBD, likely depend on the stage of skeletal maturation at disease onset and the disease effects on the periosteal and endosteal surfaces. Children further from peak bone mass at Crohn disease onset may have irreversible deficits not seen in adult-onset Crohn disease.
Biochemical Markers of Bone Metabolism
Biochemical markers of bone metabolism are released into the circulation during the process of bone formation and resorption, providing information about the dynamic process of bone metabolism. Biomarkers of formation, such as bone-specific alkaline phosphatase, are by-products of osteoblast activity. Biomarkers of bone resorption are related to collagen degradation products, including pyridinium cross-links and C-telopeptide of collagen cross-links (β-CTX) [21]. In adults, biochemical markers of bone turnover correlate well with formation and resorption, as measured by bone biopsy, and are independent predictors of fracture risk [22]. Further, bone biomarkers can be used to monitor the effectiveness of bone therapies [9]. Because formation and resorption are tightly coupled in adults, drugs that increase bone formation (e.g., teriparatide) increase markers of formation and resorption, while drugs that inhibit resorption (e.g., bisphosphonates) decrease markers of formation and resorption [23].
In adults, bone metabolism is primarily due to remodeling. However, in children biomarkers of bone metabolism represent the aggregate turnover due to (1) endochondral bone formation (longitudinal growth of bone), (2) increase in bone circumference, and (3) bone remodeling [24]. The pubertal growth spurt is reflected by marked increases in bone biomarkers [25]. Therefore, use of bone biomarkers in children and adolescents requires consideration of gender, pubertal maturation and growth velocity; [25] and is most appropriately limited to short-term longitudinal studies to assess the impact of specific interventions [24].
Potential Threats to Bone Health in Pediatric IBD
Osteopenia has been well-documented in children and adults with IBD [26–30]. We, and others, have reported vertebral compression fractures with children with IBD, [3–5] and hip, spine, and forearm fracture rates are significantly increased in adults with Crohn disease [31–36]. Kappelman et al. found that children with IBD < 12 years of age had an increased risk of fracture (odds ratio [OR] 2.2, 95% confidence interval [CI] 1.2–3.8) and children with CD had a trend toward an increased risk of vertebral compression fracture, both, compared with controls [7]. The osteopenia in IBD is multifactorial; likely etiologies include growth failure, delayed maturation, anorexia, malabsorption, cytokine effects on bone cells, and glucocorticoid therapies.
Malnutrition
Children with IBD are at risk for inadequate intake of calories as well as micronutrients including calcium, vitamin D and zinc secondary to anorexia due to upper tract inflammation, malabsorption, increased metabolic demands, lactose intolerance, abdominal pain, or depression. Even in the setting of adequate caloric intake, malabsorption will cause deficiency states of the above micronutrients depending on location and severity of disease. Diarrhea can result in zinc deficiency which impacts on growth. Vitamin D deficiency may result from malabsorption as well as decreased exposure to sunlight due to disease flares. Vitamin K deficiency may result from malabsorption and altered bowel flora due to antibiotic use and results in increased concentrations of undercarboxylated osteocalcin which is associated with decreased bone turnover and fractures [37]. Nutrients that may contribute to impaired bone acquisition in pediatric IBD include calcium, vitamin D, vitamin K, and magnesium [38].
Multiple studies have reported that vitamin D deficiency frequently complicates pediatric IBD [39–42]. For example, Pappa et al. recently examined vitamin D levels in 130 children and young adults with IBD, 94 with Crohn disease and 36 with ulcerative colitis. The prevalence of vitamin D deficiency [serum 25 (OH) vitamin D concentration ≤ 15 ng/mL] was 34.6%, and the mean serum 25 (OH) vitamin D concentration was similar in patients with Crohn disease and ulcerative colitis, 52.6% lower among patients with dark skin complexion, 33.4% lower during the winter months (December 22–March 21), and 31.5% higher among patients who were taking vitamin D supplements. Patients with Crohn disease and upper gastrointestinal tract involvement were more likely to be vitamin D deficient than those without it. A similar study reported that 45% of children with IBD had vitamin D levels less than 20 ng/mL [39]. Of note, none of these studies detected a relation between vitamin D levels and spine BMD, as measured by DXA [39–41].
Decreased Muscle Mass and Biomechanical Loading of the Skeleton
Bone adapts its strength in response to the magnitude and direction of the forces to which it is subjected. Mechanical forces on the skeleton arise primarily from muscle contraction. This capacity of bone to respond to mechanical loading with increased bone size and strength is greatest during growth, especially during adolescence [43]. Numerous studies have documented the beneficial effect of physical activity and biomechanical loading on bone geometry in healthy children [44–49]. These relationships dictate that studies of bone health in chronic childhood diseases consider the effects of alterations in muscle mass and strength.
Weight-bearing physical activity and biomechanical loading of bone are critical determinants of bone mass in growing normal children [50]. The influence of skeletal loading on bone accretion is illustrated in two exercise trials in healthy children. An easily implemented school-based jumping intervention augmented cortical thickness in the femoral neck of healthy children [51]. A randomized clinical trial of physical activity and calcium supplementation in prepubertal children resulted in a significant, positive interaction between calcium supplements and physical activity in both cortical thickness and cortical area [52]. Harpavat et al. recently reported that none of the subjects in a small series of children with IBD were participating in weight-bearing physical activities [53]. To our knowledge, no studies have compared physical activity patterns in children with IBD and healthy controls. Nonetheless, the reports of decreased lean mass in pediatric IBD suggest that decreased biomechanical loading of the skeleton may contribute to impaired bone accrual in this disorder.
The relations between bone and muscle mass have been demonstrated in multiple studies in children and adolescents with Crohn disease. Burnham et al. reported that Crohn disease was associated with a 0.50 SD deficit (p = 0.006 compared with controls) in whole body bone mineral content (BMC) relative to height in males, adjusted for age, race, and Tanner stage [8]. Adjustment for whole body lean mass attenuated this deficit to 0.19 SD (p = 0.13 compared with controls). The authors noted that the absence of a bone deficit after statistical adjustment for lean mass does not imply that the bones are normal or adequate. In a similar study, deficits in DXA estimates of femoral neck subperiosteal width were not statistically significant after adjustment for lean mass [54]. Finally, a recent prospective cohort study using tibia peripheral QCT in children with newly diagnosed Crohn disease reported that muscle mass improved significantly over 1 year following diagnosis, but section modulus (a summary measure of cortical bone dimensions and strength) worsened significantly [55]. This apparent disconnect between changes in bone and muscle mass over time illustrates the limitations of the functional muscle bone unit paradigm in chronic inflammatory disease.
Glucocorticoid-Induced Osteopenia
Glucocorticoids are widely used in the treatment of IBD and impact bone formation and resorption. Decreased bone formation is the primary mechanism for bone loss in glucocorticoid-induced osteopenia [56]. Mesenchymal stems cells, which also give rise to adipocytes, myoblasts and chondrocytes, differentiate into osteoblasts. Glucocorticoids shift the cellular differentiation away from osteoblasts and toward adipocytes, and prevent the termination differentiation of osteoblasts [57]. Osteoblast numbers are decreased further by glucocorticoid-induced increases in osteoblast apoptosis [58]. In addition, glucocorticoids inhibit osteoblast production of bone matrix components [59]. Finally, glucocorticoids suppress the synthesis of insulin-like growth factor-I (IGF-1), an agent that enhances bone formation [60]. The cellular response to glucocorticoids also includes an early phase of increased bone resorption, probably a result of the increased expression of receptor activator of nuclear factor-κB ligand (RANKL) and decrease osteoprotegerin (OPG)—increased RANKL and decreased OPG both promote osteoclastogenesis, as detailed below [61]. However, typically a more chronic state of decreased bone resorption develops, due to loss of cell signaling to osteoclast progenitors [62].
Patients treated with glucocorticoids have an underlying disease which frequently also carries a risk of osteoporosis. Therefore, the independent effects of glucocorticoids on bone turnover and bone structure during growth are not readily apparent from clinical studies. However, recent animal models demonstrate that glucocorticoid administration during growth resulted in decreased bone formation, decreased bone resorption, reductions in the age-dependent increases in trabecular thickness, and reductions in linear growth and accrual of cortical thickness in the femur [63, 64]. These deficits were associated with decreased bone strength in the vertebrae and femur in mechanical testing [63, 64]. Of note, it is unclear if the reductions in femoral cortical thickness were proportionate to the significant reductions in bone length. That is, did the bones have normal cortical thickness and strength relative to the shorter length?
Inflammation and Bone Loss
Cellular inflammatory pathways in Crohn disease activate the protean transcriptional regulatory factor nuclear factor-κB with increased production of a variety of cytokines, such as interleukin-6 (IL-6) and tumor necrosis factor-α (TNF-α) [65]. Three groups of cytokines are particularly important in bone physiology: interleukin-6 (IL-6), TNF-α, and lL-1 [61]. Inflammatory cytokines promote osteoclastogenesis and accelerated bone resorption. TNF-α induces the expression of receptor activator of NF-κB ligand (RANKL). RANKL stimulates osteoclast differentiation and activation and inhibits osteoclast apoptosis, thereby dramatically prolonging osteoclast survival and increasing bone resorption [66, 67]. Additionally, TNF-α decreases expression of OPG, a decoy receptor that blocks RANKL [68, 69]. Inflammatory mediators, including IL-1 and IL-6, also increase RANKL secretion and contribute to bone loss [70]. TNF-α also has direct effects on bone formation; it inhibits osteoblast differentiation, inhibits osteoblast collagen secretion, causes increased resorption by inducing osteoblasts secretion of IL-6, and induces osteoblast apoptosis [71, 72]. These effects on bone formation are strikingly similar to the effects of glucocorticoids [56, 57].
Assessment of Bone Status in Children and Adolescents
Classification of Bone Health and Relation to Fracture Risk
DXA is widely accepted as a quantitative measurement technique for assessing skeletal status. In elderly adults, DXA BMD is a sufficiently robust predictor of osteoporotic fractures that it can be used to define the disease. The World Health Organization criteria for the diagnosis of osteoporosis in adults is based on a T-score, the comparison of a measured BMD result with the average BMD of young adults at the time of peak bone mass [73]. A T-score ≤ − 2.5 SD below the mean peak bone mass is used for the diagnosis of osteoporosis, and a T-score ≤ − 2.5 SD with a history of a low-impact fracture is classified as severe osteoporosis. While the T-score is a standard component of DXA BMD results, it is clearly inappropriate to assess skeletal health in children through comparison with peak adult bone mass. Rather, children are assessed relative to age or body size, expressed as a Z-score. In adults, low impact fractures are defined as fractures that occur after a fall from standing height or less. This definition is often difficult to apply to fractures in children that occur during play or sports activities.
Despite the growing body of published normative data utilizing DXA in children, there are no evidence-based guidelines for the definition of osteoporosis in children. Fractures occur commonly in otherwise healthy children with a peak incidence during early adolescence around the time of the pubertal growth spurt [21]. Faulkner et al. recently reported that peak gains in bone area preceded peak gains in BMC in a longitudinal sample of boys and girls, supporting the theory that the dissociation between skeletal expansion and skeletal mineralization results in a period of relative bone weakness [74]. This may be due to increased calcium demands during maximal skeletal growth. Khosla et al. recently reported that forearm (the most common site) fracture rates have increased significantly in males and females over the last 30 years; the peak incidence and greatest increase occurred between ages 11 and 14 years in boys and 8 and 11 years in girls [21]. Possible explanations include changing patterns of physical activity or decreased bone acquisition due to poor calcium intake.
Several studies have compared the DXA BMD of normal children and adolescents with forearm fractures to that of age-matched controls without fractures. Most [75–79], but not all [80, 81], found that mean DXA BMD was significantly lower in children with forearm fractures than in controls. One study reported that 69% of fractures in healthy children were due to low-energy falls at home; [80] illustrating the difficulties defining low-energy fractures in children. Studies using QCT or metacarpal morphometry to characterize cortical geometry showed that decreased cortical thickness was associated with significantly increased fracture risk [79, 82]. Finally, television, computer, and video viewing had a dose-dependent association with wrist and forearm fractures [83]. A recent prospective cohort study in over 6,200 children in the United Kingdom reported a weak inverse relationship between whole body (less head) BMD at 9.9 years of age and subsequent fracture risk [odds ratio (OR) per SD decrease = 1.12; 95% CI, 1.02–1.25] [84]. The association between fracture risk and BMD was much stronger when adjusted for bone and body size, fracture risk was inversely related to BMC adjusted for bone area, height, and weight (OR = 1.89; 95% CI, 1.18–3.04).
These data suggest that low DXA BMD can be a contributing factor for pediatric fracture in healthy children; however, bone geometry and nonskeletal factors such as sports participation, body size, and sedentary activities contribute to fracture risk. Importantly, the relationships between DXA BMD, bone geometry and fracture risk in children with chronic diseases, such as IBD, may be different that those observed in healthy children.
Limitations of DXA in Children and Adolescents
DXA is, by far, the most commonly employed method for the assessment of bone health in children. However, DXA has several limitations that are pronounced in the assessment of children (Table 23.1). A recent study highlights the importance of these limitations [85]: among children referred for enrollment in a childhood osteoporosis protocol based on low DXA spine BMD, 80% had at least one error in interpretation of the DXA scan. Ultimately, only 26% retained the diagnosis of low BMD.
Table 23.1
Limitations of DXA techniques in infants and children
Scan acquisition |
Fan beam results in magnification error with apparent differences in bone area and BMC as body size varies [161] |
Scan analysis |
Difficult to define landmarks and region of interest in the immature hip [162] |
Limited data in young children | |
Analysis methods not standardized | |
Variable hardware and software across published reference data sets | |
Some are not gender-specific [107] | |
Some presented relative to age, others relative to height, Tanner stage, and weight | |
Interpretation |
|
Unable to distinguish between changes in bone dimensions and density | |
Unable to distinguish between cortical and trabecular bone |
The significant limitation of DXA is the reliance on measurement of areal rather than volumetric BMD. DXA provides an estimate of BMD expressed as grams per anatomical region (e.g., individual vertebrae, whole body, or hip). Dividing the BMC within the defined anatomical region (g) by the projected area of the bone (cm2) then derives “areal-BMD” (g/cm2). This BMD is not a measure of volumetric density (g/cm3) because it provides no information about the depth of bone. Bones of larger width and height also tend to be thicker. Since bone thickness is not factored into DXA estimates of BMD, reliance on areal-BMD inherently underestimates the bone density of short people. Despite identical volumetric bone density, the child with smaller bones appears to have a mineralization disorder (decreased areal-BMD). This is clearly an important artifact in children with chronic diseases, such as IBD, that are associated with growth delay and short stature [86].
The confounding effect of skeletal geometry on DXA measures is now well recognized and multiple analytic strategies have been proposed to express DXA bone mass in a form that is less sensitive to differences in skeletal size [87–91]. The technique developed by Carter et al. is based on the observation that vertebral BMC scaled proportionate to the projected bone area to the 1.5 power [87]. Therefore, vertebral volume is estimated as (area)1.5 and bone mineral apparent density (BMAD) is defined as BMC/(area)1.5. Kroger et al. proposed an alternative estimate of vertebral volume: the lumbar body is assumed to have a cylindrical shape and volume of the cylinder is calculated as (π)(radius2)(height), which is equivalent to (π)((width/2)2)(area/width) [92, 93]. This approach was validated by comparison with MR measures of vertebral dimensions in 32 adults; [88] DXA-derived volumetric BMD correlated moderately well with BMD based on MR-derived estimates of vertebral volume (R = 0.665). Although these methods provide estimates of vertebral volume, the BMC includes the bone content of the superimposed cortical spinous processes.
A recent study by Wren et al. sought to evaluate the usefulness of DXA spine correction factors based on published geometric formula and anthropometric parameters, compared with three-dimensional QCT [94]. Subject height, weight, body mass index (BMI), skeletal age, and Tanner stage were assessed in 84 healthy children. Two geometric calculations based on DXA spine results were used to estimate volumetric BMD: (1) BMAD and (2) areal BMD/bone height. Linear regression was used to compare DXA–BMC vs. QCT–BMC and CT volumetric BMD vs. DXA areal BMD, BMAD and (areal BMD/bone height). DXA and QCT BMC were highly correlated (r 2 = 0.94). However, DXA areal BMD correlated more strongly with CT Vol (r 2 = 0.68) than with CT density (r 2 = 0.39), illustrating the confounding effect of bone size on DXA areal BMD results. The use of DXA correction factors only slightly improved the density correlations [r 2 = 0.49 for BMAD; r 2 = 0.55 for (areal BMD/bone height)]. The correlations between QCT volumetric BMD and DXA estimates were particularly poor for subjects in Tanner stages 1–3 [r 2 = 0.02 for areal BMD; r 2 = 0.13 for BMAD; r 2 = 0.27 for (areal BMD/bone height)]. In contrast, multiple regression accounting for the anthropometric and developmental parameters greatly improved the agreement between the DXA and CT densities (r 2 = 0.91). These results suggest that DXA BMC is a more accurate and reliable measure than DXA BMD for assessing bone acquisition, particularly for prepubertal children and those in the early stages of sexual development. Use of DXA BMD would be reasonable if adjustments for body size, pubertal status, and skeletal maturity are made, but these additional assessments add significant complexity to research studies, and to clinical interpretation.
An additional shortcoming of DXA is that the integrated measure of bone mass in a given projected area does not allow distinction between cortical and trabecular bone. DXA-based measures provide no information on bone architecture, and are limited in their usefulness to differentiate the spectrum of bone accrual during growth.
Comparisons to appropriate pediatric reference data are essential to describe accurately the clinical impact of childhood disease on bone development, to monitor changes in bone mineralization, and to identify patients for treatment protocols. Multiple sources of pediatric DXA reference data are now available for the calculation of DXA Z-scores. These include varied approaches, such as gender-specific centile curves, age- and height-specific means and standard deviations, Tanner- and weight-specific percentiles, age-, sex-, weight- and height-adjusted curves, and Z-score prediction models [89, 90, 95–106]. Differences in reference data have a significant impact on the diagnosis of osteopenia in children with chronic disease [107]. For example, use of reference data that are not gender-specific results in significantly greater misclassification of males as osteopenia [107]. In addition, use of published pediatric reference ranges has been complicated by differences in scanner manufacturers, and frequent changes in hardware and software technology, including fan-beam technology, low-density software analysis modes and specialized pediatric software. These technical changes result in clinically significant alterations in DXA results [108]. Further effort is needed to develop adequate reference data and validate classification schemes of bone health in children.
Peripheral Quantitative Computed Tomography
A three-dimensional structural analysis of trabecular architecture and cortical bone dimensions can be obtained by computed tomography (CT). This technique offers an opportunity to overcome these limitations and advance our understanding of bone mineralization in children. CT provides an image unobscured by overlying structures [109]. The CT attenuation of different bone tissues provides quantitative information, referred to as quantitative CT (QCT). In contrast to DXA, this technique describes authentic volumetric BMD (vBMD), accurately measures bone dimensions, and distinguishes between cortical and trabecular bone. In order to minimize radiation exposure, special high-resolution scanners were developed for the peripheral skeleton (pQCT), specifically, the radius or tibia. The distal site is largely trabecular bone, while the mid-shaft is almost entirely cortical bone. The volume of each component is calculated from the scan thickness and cross-sectional area, and the density by attenuation of the X-ray beam. Bone strength can also be estimated by pQCT from the total bone area, and cortical thickness and density [110]. For example, QCT studies of bone mineral accretion and bone strength demonstrated gender, maturation, and ethnic-specific patterns of development of bone strength during childhood and adolescence [111].
Clinical Studies of Bone Health in Pediatric IBD
Numerous studies have reported decreased DXA BMD in children with IBD [39]. However, as detailed above, DXA studies are frequently confounded by disease effects of growth. For example, two recent studies reported that DXA BMD for age Z-scores were significantly correlated with height for age Z-scores in children with IBD [39, 112]. Furthermore, expression of DXA results as BMAD (an estimate of volumetric BMD) eliminated the correlation with height Z-scores [112]. Another study addressed the confounding effect of short stature by expressing the spine DXA results as percent predicted BMC for bone area for age and gender in 73 children with Crohn disease or ulcerative colitis [113]. The percent predicted bone area for age and gender was decreased in IBD, compared with controls, consistent with shorter stature. While the median BMD for age and gender Z-score was significantly decreased in IBD (mean Z-score in spine = − 1.6, in whole body = − 0.9), the percent predicated BMC for bone area, age, and gender was normal. The authors concluded that children with IBD have small bones for age due to growth retardation, but adequate bone mass relative to bone size. Finally, Herzog et al. reported that BMD Z-scores were less than −2.0 in 44% of children when expressed relative to chronologic age, but were less than −2.0 in only 26–30% when expressed relative to bone age of height age [114].
The above studies illustrate the varied approach used to adjust for the confounding effects of poor growth. We recently reported that whole body BMC relative to height predicted cortical bone CSMI (an estimate of bone strength, as described above) as measured by QCT [91]. Therefore, we assessed whole body BMC, lean mass and fat mass (as measured by DXA) relative to height in 104 children and young adults with established Crohn disease, and 233 healthy controls, 4–26 years of age. The studies demonstrated significant bone and muscle deficits [8, 115]. Individuals with Crohn disease had significantly lower height-for-age, BMI-for-age, and whole body lean mass-for-height Z-scores than healthy controls (all p < 0.001). Ninety percent of Crohn disease subjects had been treated with glucocorticoids. The cumulative exposure averaged 7,900 mg over 15.2 months, resulting in an average dose of 0.50 mg/kg/day. Table 23.2 summarizes four sequential models in males and females. The least adjusted models assessed whole body BMC in Crohn disease, compared with controls, adjusted for age and race, and revealed substantial deficits. Assessment of BMC without consideration of the decreased skeletal size for age in subjects with Crohn disease group may overestimate bone deficits. Accordingly, the second model was also adjusted for height. Fig. 23.3 demonstrates that the marked BMC deficits relative to age (a) are less pronounced when assessed relative to height (b). Adjustment for height attenuated the Crohn disease effect in the multivariate regression model; however, significant BMC deficits persisted in males and females with Crohn disease, compared with controls. In order to determine if delayed pubertal maturation for age contributed to the decreased BMC in Crohn disease, the third model in Table 23.2 included Tanner stage. Adjustment for delayed pubertal maturation did not appreciably change the estimate of BMC deficits in Crohn disease. The fourth and final model, adjusted for lean mass, eliminated significant BMC deficits in Crohn disease.
Table 23.2
Hierarchical models of whole body BMC Z-scores in Crohn disease [8]
Models |
Males |
Females | ||
---|---|---|---|---|
Z (95% CI) |
p |
Z (95% CI) |
p | |
Age, race |
−1.16 (−1.51, −0.82) |
< 0.001 |
−0.61 (−0.95, −0.27) |
0.001 |
Height, age, race |
−0.63 −0.95, −0.30) |
< 0.001 |
−0.44 (−0.81, −0.06) |
0.02 |
Height, age, race, tanner |
−0.50 (−0.85, −0.15) |
0.006 |
−0.35 (−0.72, 0.02) |
0.06 |
Height, age, race, tanner, lean mass |
−0.19 (−0.43, 0.06) |
0.13 |
−0.05 (−0.34, 0.25) |
> 0.2 |
Table 23.3
Bisphosphonate studies in children and adolescents with chronic inflammatory disease
Study/subjects |
Intervention/outcome |
Comments and results |
---|---|---|
Bianchi et al. [167]
Chronic rheum disorder and ↓ spine BMD
N = 39a, age 5–18 |
Design: 12 month case-series
Oral alendronate
Weight ≤ 20 kg: 5 mg q day
Weight > 20 kg: 10 mg q day
Instructed to ↑ calcium to RDA
Outcome: DXA spine areal-BMD |
Serum alkaline phosphatase levels decreased by 16.5 ± 10.8%
Urinary excretion of NTX decreased by 17 ± 16.5%
Mean spine areal-BMD Z-scores (adjusted for sex, age, body surface area) increased from a mean of −2.7 at baseline to −1.9 at 6 months (p < 0.01 compared with baseline) and to −1.05 at 12 months (p < 0.001 compared with baseline) |
Rudge et al. [11]
Chronic glucocorticoids
N = 22b, age 4–17 |
Design: 12 month RCT
Oral alendronate vs. placebo
1–2 mg/kg weekly
No calcium supplements
Rx Vit D if level <20 ng/mL
Outcome: DXA of spine and femur shaft |
Baseline height Z-score significantly greater in placebo group (−0.2 vs. −2.0)
18 completed study
Significant ↓ in bone resorption markers in alendronate group (p < 0.01)
Lumbar spine: significant ↑ in BMAD in alendronate group (p = 0.013) compared with baseline, but not in placebo group (p = 0.16)
Femur mid-shaft: marginal ↑ in CSMI in alendronate group (p = 0.08) compared with baseline, but not in placebo group (p = 0.18) |

Fig. 23.3
Distribution of whole body BMC relative to age (a) and relative to height (b) in children and young adults with Crohn disease, compared with healthy controls. (From Burnham et al. [8], with permission from John Wiley & Sons)
None of the glucocorticoid measures were significantly correlated with BMC-for-height Z-scores. However, height Z-score was negatively and significantly associated with duration of glucocorticoid therapy (r = − 0.24, p = 0.02), and cumulative (mg/kg) glucocorticoids (r = − 0.36, p < 0.001). Parenteral nutrition, isolated upper tract disease, hypoalbuminemia, nasogastric feeding, and decreased BMI Z-scores were associated with decreased BMC-for-height Z-scores.
Over 90% of the children and young adults in the prior study had a history of glucocorticoid exposure; therefore, it was not possible to distinguish between disease and glucocorticoid effects on bone. The impact of the underlying IBD process is best assessed is subjects with newly-diagnosed disease. The largest study of DXA BMD in newly diagnosed subjects was reported by Gupta et al [116]; however, the study was complicated by the observation that BMD was markedly decreased in controls, compared with the DXA reference database. Overall, DXA spine BMD was comparable in the 41 children with ulcerative colitis and the controls, while results were significantly lower in the 82 subjects with Crohn disease.
Sylvester recently examined DXA BMD and bone biomarkers in 23 children with newly diagnosed Crohn disease [117]. Although BMD Z-scores did not differ between Crohn disease subjects and controls in this small sample, bone biomarkers were significantly lower in Crohn disease. This may be due to reduced bone remodeling, or reduced growth velocity. Importantly, activated T cells produced greater concentrations of interferon-γ, which may contribute to lower bone turnover. Another small series in 18 children with newly diagnosed Crohn disease demonstrated that BMD was below the 16th percentile for age in 28% of subjects [53]. Delayed pubertal development correlated with lower BMD Z-scores, which may represent the confounding effect of poor growth.
Walther et al. recently compared lumbar spine BMAD Z-scores in 34 steroid-naïve and 53 steroid-treated children with IBD in order to obtain information about the influence of nonsteroidal factors [112]. Overall, 56 had Crohn disease and 30 had ulcerative colitis. Reference data were obtained in 52 controls. The mean BMAD Z-scores in the subjects with Crohn disease were −0.76 ± 1.25 in females and −0.79 ± 0.92 in males. The mean BMAD Z-scores in the subjects with ulcerative colitis were −0.30 ± 0.75 in females and −1.08 ± 1.23 in males. Among the steroid-naïve subjects, the duration of treatment ranged from 0 to 8 years; the majority (approximately 80%) were within the first 5 weeks of therapy. Among the steroid-treated subjects, the cumulative steroid exposure averaged 4,600 mg (range 0.05–25,000 mg) over the treatment duration of several days to 7.6 years. The mean BMAD Z-scores were comparable in steroid-naïve (−0.74 ± 1.08) and steroid-treated (−0.66 ± 1.08) subjects. The 19 subjects that had been treated with calcium and/or vitamin D supplements were all within the steroid-treated group. The study is limited by the small number of controls, and the lack of data on disease activity between the steroid-naïve and steroid-treated groups. Nonetheless, these data indicate bone deficits in the absence of steroid therapy. The studies listed above were all based on DXA estimates of BMC and BMD, and did not distinguish between cortical and trabecular bone. Dubner et al. performed tibia pQCT in 78 CD subjects (ages 5–18 year) at diagnosis and followed them for 12 months [55]. At diagnosis, CD subjects had significant deficits in trabecular vBMD (Z-score: −1.32 ± 1.32, p < 0.001), section modulus (a summary measure of cortical bone dimensions and strength) (−0.44 ± 1.11, p < 0.01), and muscle (−0.96 ± 1.02, p < 0.001), compared with controls. Over the first 6 months, trabecular vBMD and muscle Z-scores improved significantly (both p < 0.001); however, section modulus worsened (p = 0.0001) and all three parameters remained low after 1 year. Improvements in muscle were associated with improvements in section modulus and improvements in trabecular vBMD were greater in prepubertal subjects. Werkstetter et al. in a longitudinal study using forearm pQCT of 102 pediatric IBD patients (82 CD, 30 newly diagnosed), showed similar findings at diagnosis and median follow-up interval of 2.6 years (0.9–5.8) [118]. Finally, only one study has addressed the impact of childhood IBD on peak bone mass. Bernstein et al. assessed spine, proximal femur, and whole body BMD in 780 premenopausal adult women (age < 45 years) that were diagnosed with IBD prior to 20 years of age [119]. The mean BMD T-scores were normal in the spine (−0.14 ± 1.05), femoral neck (−0.15 ± 1.04) and whole body (0.09 ± 1.04) and results did not differ between the 12 subjects with disease onset before puberty, and the 58 subjects with disease onset after puberty.
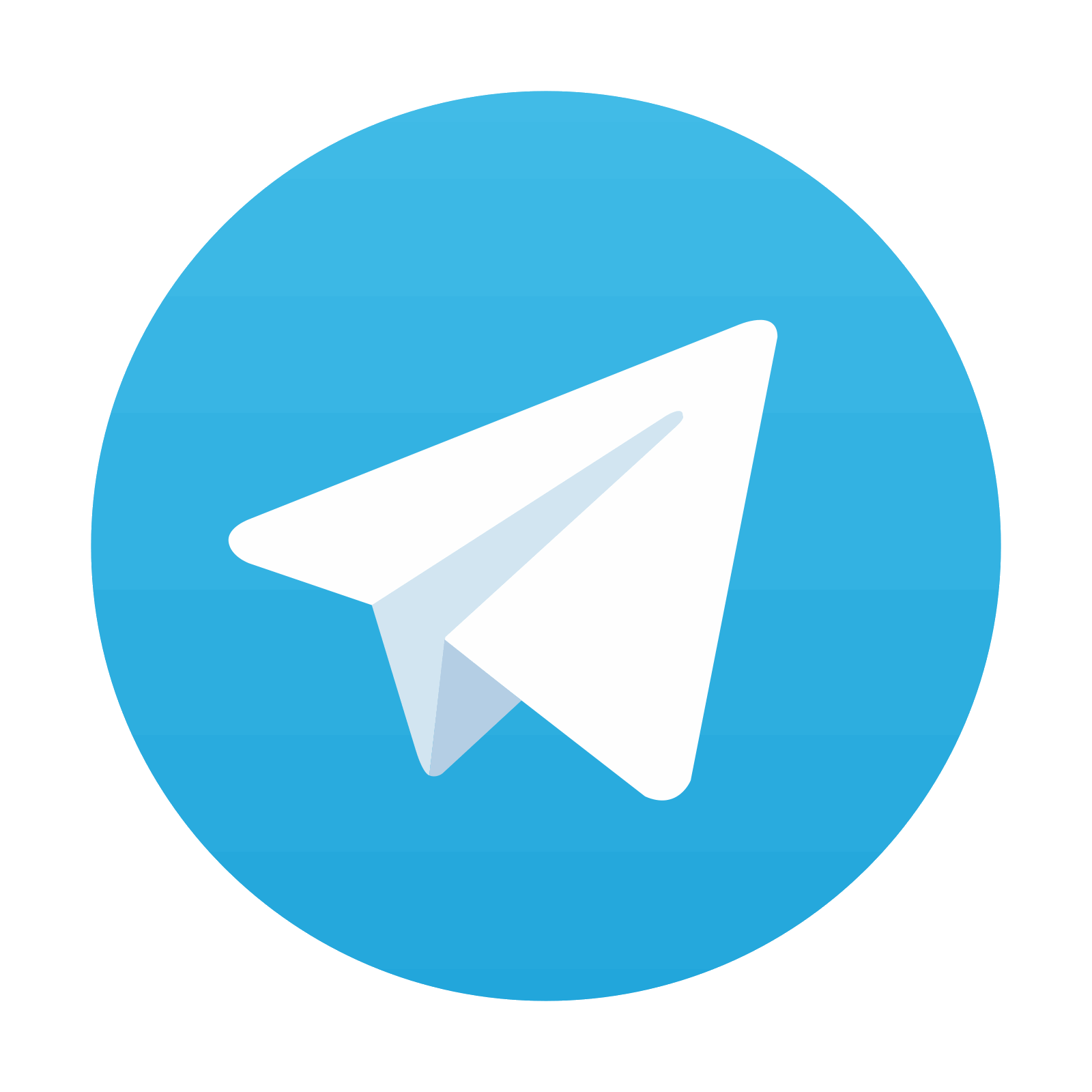
Stay updated, free articles. Join our Telegram channel
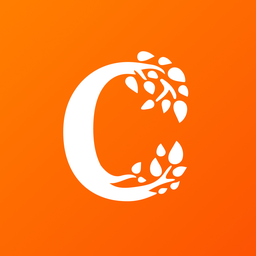
Full access? Get Clinical Tree
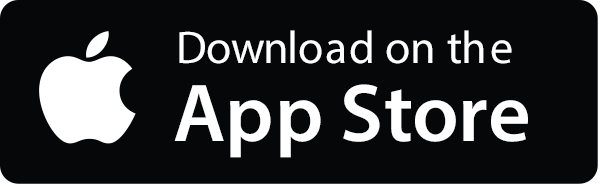
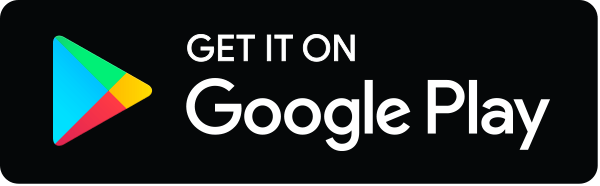