Fig. 11.1
Netrins and their receptors in the vascular system. a Netrin family members show a structural homology to the β-laminin (Netrin4) or γ-laminin (Netrin1, 2, 3, and G) subunits. b DCC and Unc5A–D are the main netrin receptors. Neogenin, DSCAM, or integrins also bind to netrins and can function in a co-receptor complex. c An angiogenic sprout with an endothelial tip cell followed by stalk and phalanx endothelial cells (ECs). Smooth muscle cells (SMCs) and pericytes cover stalk and phalanx cells. Unc5 and neogenin are expressed on sprouting ECs as well as mural cells. d Unbound Unc5B in ECs activates death-associated protein (DAP) kinase signaling that induces apoptosis. e Netrin1 binding to Unc5B in ECs inhibits apoptosis signaling and leads to the stabilization of the angiogenic sprout. Netrin1/neogenin interactions on SMCs induce their proliferation and migration, which further enhances vascular stabilization. f Netrin4 blocks angiogenesis by binding to Unc5B/neogenin heterodimers in ECs. g Netrin4/integrin signaling in lymphatic endothelial cells induces lymphangiogenesis. (Adapted with permission from Macmillan Publishers Ltd: Mehlen et al. [48], copyright 2011; Cirulli and Yebra [174], copyright 2007; Kitajewski [175], copyright 2011)
Netrin1 is the best-studied member of the netrin family. It guides different neuronal populations to distinct locations, and controls central nervous system (CNS) development [15–21]. One of the best-known roles for Netrin1 is in the developing spinal cord, where floor-plate-derived Netrin1 attracts ventral-directed commissural axons and regulates their midline crossing [4]. Netrin4 and NetrinGs are also found in the CNS, and also in non-neuronal tissue [22, 23]. Functionally, Netrin4 was found to promote neurite outgrowth in cortical explants, whereas NetrinG1 induces the outgrowth of thalamocortical axons via NGL1 binding [1, 5, 24].
11.2.2 Netrins and Netrin Receptors in the Vascular System
Expression of Netrin Receptors in Endothelial Cells
In addition to the nervous system, there is accumulating literature showing a potent effect of netrins during vascular development and angiogenesis. Netrin1 and Netrin4 are the best-studied members, whereas a functional role of Netrin3 and NetrinGs in the vascular system lacks robust investigations. Interestingly, similar to what occurs in neurons, the effect of Netrin1 and Netrin4 stimulation in the vascular system depends on the EC origin and is linked to differential netrin receptor expression. Generally, ECs do not express DCC but express low levels of Unc5B, C, and D, as well as neogenin [25–29] (Fig. 11.1c). However, human arterial ECs (HUAECs) express higher levels of Unc5B than human umbilical vein ECs (HUVECs) [one of the most commonly used cells for studying angiogenesis] [27]. This higher expression of Unc5B in arterial ECs was also shown in vivo in the developing mouse retina where Unc5B expression was detected in ECs of arteries but not veins [27]. Apart from the basal expression levels, Unc5 receptor expression in ECs seems to be regulated by angiogenic factors such as vascular endothelial growth factor-A (VEGFA, hereon termed VEGF) [30].
Function of Unc5B and Netrin1 in Physiological Angiogenesis
Two different vascular phenotypes have been described in Unc5B mutant mice. On the one hand, it has been shown that Netrin1-induced Unc5B activation in ECs led to an antiangiogenic effect similar to the repulsive effect observed in the nervous system. Genetic Unc5B depletion led to increased, but collapsed, arterial branches accompanied by abnormal endothelial tip-cell navigation and extensive filopodia extension in different vascular beds of the mouse embryo (in the CNS vasculature and the retina), as well as in intersegmental vessels of Unc5B morphant zebrafish [27]. These results suggest that, in the absence of Unc5B, there is reduced repulsion of angiogenic blood vessels by Netrin1. Similarly, studies in chicken embryos show that Netrin1 inhibits angiogenic sprouting of Unc5B-positive vessels [31]. Netrin1 stimulation of HUAECs in vitro also results in a Unc5B-dependent reduction of migration and increased filopodia retraction [27].
On the other hand, in vitro and in vivo experiments by Li’s group showed that Netrin1 positively stimulated angiogenesis and that the knockdown of Netrin1 in zebrafish resulted in inhibition of the parachordal vessel, rather than in increased vascular branching [28, 29]. Moreover, analysis of another Unc5B mutant mouse line revealed normal blood vessel branching in the hindbrain, cranial, and intersomitic vessels [27]. The generation of an EC-specific Unc5B mutant mouse line showed that endothelial specific ablation of Unc5B causes embryonic lethality and that Unc5B is required for proper development of the vascular bed of the placenta but not the hindbrain or yolk sac, which appeared normal [32].
Consistent with an angiogenic role of Netrin1, different EC lines such as mouse brain ECs, human microvascular ECs (HMVECs), human aortic endothelial cells (HAECs), or HUVECs showed a positive angiogenic response to Netrin1 stimulation with increased sprouting, proliferation, migration, and tube-formation; however, no known netrin receptors could be identified in these cell lines [25, 28, 29, 33]. Interestingly, vascular smooth muscle cells (SMCs) express neogenin (Fig. 11.1c), and Netrin1 stimulation in vitro leads to a positive effect on SMC adhesion, proliferation, and migration (Fig. 11.1d) [28]. Moreover, in vivo experiments such as xenografts, chicken chorioallantoic membrane (CAM) assays, and corneal pocket assays result in increased angiogenesis in response to Netrin1, as well as in a synergistic effect together with VEGF [25, 28].
As mentioned previously, Unc5 members and DCC are known to function as dependence receptors, which can be activated both in the presence and absence of their ligands. In the absence of Netrin1, DCC and Unc5 unbound receptors activate a proapoptotic cascade, leading to cell death via activation of caspases [34]. In this regard, during zebrafish development, Netrin1 prevents ECs from apoptosis in a Unc5B-dependent manner, whereby genetic knockdown of Netrin1 leads to increased cell death of ECs and vascular defects that can be rescued by Unc5B inhibition [35]. Netrin1 inhibits in vitro Unc5B downstream caspase signaling and decreases the level of apoptosis in HUAECs and HUVECs that could be mimicked by Unc5B silencing [35] (Fig. 11.1d, e). Netrin1/Unc5B signaling leads to inactivation of the death-associated protein kinase (DAPK) and thus blockage of apoptosis [35] (Fig. 11.1d). Regarding DCC, to date only one study has found a role for DCC in ECs where Netrin1 induces EC proliferation and migration in vitro via activation of DCC in aortic ECs and an increase in nitric oxide production [36]. No link between DCC and its dependence function has yet been described.
Apart for acting directly on ECs, Netrin1 can also control lymphangiogenesis (the formation of lymphatic vessels) via an interplay between muscle pioneers, motoneuron axons, and ECs [37].
Altogether, it is clear that Netrin1 and Unc5B are important for physiological angiogenesis and for proper formation of the developing vasculature. Future studies will be required to better understand their functions (i.e. survival, proangiogenic, or antiangiogenic) within specific vascular beds and whether a pro- or antisurvival function can result in a pro- or antiangiogenic effect.
Unc5B and Netrin1 in Pathological Angiogenesis
A role for Netrin1 and Unc5B has also been identified in pathological angiogenesis using different disease mouse models. After postnatal angiogenesis, Unc5B becomes downregulated and remains absent in quiescent ECs [38]. However, Unc5B becomes re-expressed in adult ECs of sprouting vessels upon subjecting adult mice to oxygen-induced ischemic retinopathy (OIR). Netrin1 also becomes upregulated in the retina upon OIR, and intravitreal lentivirus delivery of Netrin1 small hairpin RNA (shRNA) inhibited retinal neovascularization, indicating Netrin1 as a potential target for different retinopathies or other ocular neovascular diseases [39].
Unc5B is also re-expressed in sprouting vessels after matrigel or tumor implants [38]. In these two experimental models, Netrin-1, via Unc5B, leads to repulsion of ECs and, as a consequence, to inhibition of matrigel neovascularization or inhibition of tumor angiogenesis (using Netrin1-containing matrigel plugs or tumor cells expressing Netrin1) [38]. However, another study showed that angiogenesis in matrigel plugs containing Netrin1 was enhanced compared with control plugs [25] (Table 11.1).
In a mouse model of hindlimb ischemia, where the iliac artery was ligated, intramuscular delivery of Netrin1 or Netrin4 into the ischemic gastrocnemius resulted in improved hindlimb perfusion and greater vascular density in Netrin1-injected muscles when compared with control injected muscles [29]. In a rat model of limb ischemia, it was consistently shown that transplantation of mesenchymal stem cells (MSCs), together with Netrin1, directly into the muscle of ischemic limbs, improves the effectiveness of MSCs and increases the neocapillary network [40]. Notably, in another study, hindlimb ischemia in adult mice (in this case ligating the femoral artery) did not lead to re-expression of Unc5B in sprouting ECs, thus suggesting that the observed improvement in the study described above might be due to Netrin1 signaling in a Unc5B-independent manner [38]. Altogether, these studies suggest Netrin1 as a possible candidate for therapeutic neovascularization. In addition, Netrin1 was found to be upregulated in patients with proliferating diabetic retinopathy (PDR), which is characterized by vascular malformations [41].
Role of Netrin4 in Physiological and Pathological Angiogenesis
Although little is known about Netrin4 signaling in the nervous system, its contribution in the vascular context has been considerably investigated; however, its signaling mechanisms still remain intricate.
Angiogenic ECs in zebrafish express Netrin4, and genetic knockdown in zebrafish using morpholinos results in vascular defects, suggesting a proangiogenic effect for Netrin4 in developmental angiogenesis [42]. Similar to Netrin1, Netrin4 stimulation increased proliferation, migration, survival, and tube formation in several EC lines, including HUVECs, HMVECs, and HUAECs in vitro [29, 42, 43]. However, the receptor mediating these proangiogenic effects remains unclear. In contrast, another study proposed an antiangiogenic role for Netrin4. In this study, it was described that Netrin4 binds neogenin, and that a neogenin/Unc5B heteroreceptor complex mediates the in vitro antiangiogenic effects of Netrin4 [26] (Fig. 11.1f). ECs of lymph vessels also express Netrin4. In vitro experiments using human lymphatic ECs revealed the same promoting effects on proliferation, migration, survival, and tube formation as with Netrin1 [44]. Although lymphatic ECs express Unc5B and neogenin, their single- or dual-receptor knockdown was insufficient to block the cellular response to Netrin4 [44]. A later study identified integrin α6β1 as a possible Netrin4 receptor in lymphatic ECs, showing the ability to bind Netrin4 (Fig. 11.1g). Consistently, the blockage of either subunit was also able to abolish the lymphatic EC migration, adhesion, and signaling induced by Netrin4 in vitro [45].
Several in vivo applications support the proangiogenic capacity of Netrin4 in blood and lymphatic vessels under pathological conditions [44]. Netrin4 administration in different stroke and ischemia models promotes neovascularization and positively affects behavior recovery without affecting integrity of the blood–brain barrier [29, 46]. It is important to mention that stroke itself upregulated Netrin4 within the ischemic core [46]. Netrin4 overexpression in mouse skin induces lymphangiogenesis in vivo. Moreover, using Netrin4 overexpressing breast cancer models, it was shown that Netrin4 promoted tumor lymphangiogenesis and tumor metastasis by stimulating lymphatic permeability [44]. However, whether Netrin4 may act differently on ECs or lympathic ECs from different tumor entities remains to be determined. In support of this, in in vivo matrigel angiogenesis assays, Netrin4 overexpression in colon cancer cells negatively affects angiogenesis in a neogenin-dependent manner, leading to reduced tumor growth and increased tumor apoptosis [47] (Table. 11.1). Although further research is still needed to understand the effects of Netrin4 in different angiogenic pathological conditions, the studies mentioned above highlight Netrin4 as a possible therapeutic target for targeting angiogenesis and lymphangiogenesis .
In summary, netrin signaling contributes to vascular development and angiogenesis, not only in a direct fashion via acting on ECs but also in a cellular interplay via acting on SMCs. Netrins exert proangiogenic and also inhibitory effects, perhaps regulated by the receptor composition. In addition, this might differ, dependent on the tissue and the specific endothelial subset. We are only just beginning to fully unravel the signaling properties of netrins in ECs. Moreover, the roles of other netrin family members (i.e. Netrin3 and NetrinGs) still need to be evaluated.
11.3 Slits and Robo Receptors
11.3.1 Introduction
Slits are secreted molecules found throughout invertebrate and vertebrate species. There are three described genes in mammals (Slit1, 2, and 3), as well as several splice variants of all three members [48]. They contain leucin-rich repeats (LRRs), endothelial growth factor (EGF) repeats, and a laminin G-like domain (Fig. 11.2a). The known receptors for slits belong to the Robo family. In mammals, there are four Robo receptors, Robo1, 2, 3, and 4 (Fig. 11.2b), together with their described isoforms Dutt1 (for Robo1), Robo2_tv2 (for Robo2, described in zebrafish and rat), as well as Robo3.1 and Robo3.2 (for Robo3) [48]. While Robo1, 2, and 3 are similar and are mainly expressed in the nervous system, Robo4 appears to be special, showing a different molecular structure accompanied with a specific expression pattern within the vascular compartment in mammals [49–51] (Fig. 11.2a). In addition, controversy exists regarding the binding of slits to Robo4 (see below).

Fig. 11.2
Slits and Robos in the vascular system. a Different domains of slits and Robo receptors. Robo4 (and also, in some cases, Robo1) is expressed on the angiogenic endothelium and on smooth muscle cells (SMCs), but not in endothelial tip cells (see scheme of sprouting blood vessel). b Left: Robo4 in zebrafish ECs regulates actin remodeling in an Rac1 Cdc42-dependent manner. Right: Slit2 and 3 binding to Robo4 blocks vascular endothelial growth factor receptor-2 (VEGFR2)-mediated Src and focal adhesion kinase (FAK) signaling, leading to vascular stabilization. Controversies exist regarding the binding of Slit2 and 3 to Robo4. c Robo4 trans-interacts with Unc5B and suppresses vascular endothelial growth factor (VEGF) signaling via VEGFR2 by blocking VEGFR2-dependent Src kinase activation. d Robo4 interacts in cis with Robo1 to transduce Slit2 signals, leading to reduced pericyte migration and therefore increased vascular stability. (Adapted with permission from Macmillan Publishers Ltd: Mehlen et al. [48], copyright 2011; Kitajewski [175], copyright 2011)
Slit/Robo signaling is involved in different neuronal processes, such as axon guidance, axon fasciculation, and dendritic branching [52, 53]. In the developing nervous system of invertebrates and vertebrates, slits were described as exerting a repulsive effect on axons [54, 55]. It was thereby found that Slit/Robo signaling regulates the midline crossing of commissural axon projections by a mechanism that involves differential expression of Robo receptors in pre- and postcrossing axons [53, 56]. Interestingly, interactions between Robo1 and the netrin receptor DCC have been identified in commissural axons [57].
11.3.2 Slits and Robo Receptors in the Vascular System
There is a growing body of literature indicating that Slit/Robo functions are not simply restricted to axonal guidance, and do indeed also play a role in the vascular system. Since Robo4 is specifically expressed in the vascular system, the majority of studies have focused on its endothelial-specific function. We summarise the main findings below.
In situ hybridization of whole-mount mouse embryos shows that Robo4 expression starts in large axial vessels and shifts from the dorsal aorta to the intersomitic vessels and capillaries as development proceeds [50]. Robo4 is also expressed in the vasculature of adult tissues [50]. Robo4 knockout mice are fertile and viable, and show normal patterning of blood vessels in different embryonic structures, indicating that Robo4 is either dispensable for developmental angiogenesis or its absence is compensated by yet an unknown mechanism [58]. In contrast, studies in zebrafish show that Robo4 depletion via morpholinos injection, and also overexpression, leads to reduced and defective formation of intersomitic vessels via a mechanism that involves Cdc42 and Rac1 Rho GTPases [59] (Fig. 11.2b).
Slit1, 2 and 3 have been proposed as ligands for Robo4 in ECs [50, 58, 60, 61]; however, other studies challenge whether slits really bind to Robo4, raising the question of potential, still unknown, ligands or a ligand-independent activation of Robo4. Here we describe studies showing activation of Robo4 by slits , as well as studies where slits did not bind to Robo4.
Function in Blood Vessel Growth and Stabilization
Analysis of Slit3 knockout mice revealed that these mice present defects in the vascular system during embryonic development . While the vasculature of diaphragms from E15.5 wild-type mouse embryos have a uniform vessel architecture, including regular vascular patterning and branching, diaphragms from Slit3 null mice show a dramatic reduction in blood vessel density and branches [61]. Consistent with this in vivo result, in vitro experiments with HUVECs, and ex vivo experiments using the rat aortic ring assay, show that Slit3 promotes EC proliferation, migration, and motility via Robo4 signaling, and also induces remodeling of the actin cytoskeleton [61].
During the growth of a new blood vessel sprout, stalk cells follow behind the leading angiogenic endothelial tip cell and participate in maturation and lumen formation of the new growing vessel [58, 62]. Phalanx cells are the most quiescent ECs in the vessels and are covered by pericytes and form tight junctions with each other (Fig. 11.2). A detailed analysis of Robo4 in the developing retina vasculature revealed that stalk cells express Robo4, while tips cells are Robo4-negative [58] (Fig. 11.2), suggesting that Robo4 might not function as a typical guidance receptor in the endothelium but rather as factor-regulating vascular stability . In support of this concept, even though Robo4 knockout mice do not show obvious differences in vascular patterning, Robo4-deficient animals possess a higher basal level of vascular permeability [58]. Moreover, when subjecting these mice to oxygen-induced retinopathy, they respond with increased vascular leakage compared with wild-type mice [58]. Robo4 stabilizing function is strengthened by results showing that Robo4 (in this case activated by Slit2 or Slit3) inhibited VEGF-induced vascular destabilization and blocked VEGF-induced EC migration, tube formation, and permeability in vitro. These effects were due to a Robo4-dependent blockage of VEGF-induced activation of the Src-family kinases [58, 63] (Fig. 11.2b). Another study in the mouse mammary gland also found a role for Slit/Robo4 signaling in restricting VEGF function by downregulating VEGFR2 activation of Src and focal adhesion kinase (FAK) [62] (Fig. 11.2b).
Interestingly, direct binding of slits to Robo4 is questioned by structural analysis as well as functional investigations of the binding properties of slits [64–66]. In a protein–protein interaction screen to identify Robo4 binding partners, no association of slits to Robo4 was detected. In contrast, an interaction with Unc5B, a Netrin1 receptor (see above), was identified [64] (Fig. 11.2c). Robo4 and Unc5B are co-expressed in ECs, and stimulation of Unc5B-transfected porcine aorta ECs (PAECs) with the extracellular domain of Robo4 (sRobo4) led to Unc5B internalization and cell retraction. Robo4/Unc5B signaling leads to the association of Src kinase to Unc5B and its activation. Moreover, Robo4/Unc5B signaling counteracts VEGF-induced Src activation by sequestering Src away from VEGFR2 [64] (Fig. 11.2c). Consistently, the intraperitoneal injection of an antibody that specifically blocks Robo4/Unc5B interaction led to increased angiogenesis and vascular hyperpermeability in vivo. Since Unc5B is expressed on all cells of the angiogenic sprout (see above), and the expression of Robo4 is excluded from the tip cell, their trans-interaction might represent an additional mechanism regulating tip and stalk cells and, consequently, the angiogenic sensitivity of the vascular sprout (Fig. 11.2c).
Altogether, the studies described above clearly demonstrate the function of Robo4 signaling for vascular stabilization, either by acting as a ligand for Unc5B or by acting as a receptor for slits. These two possible scenarios are not exclusive when considering that other studies have shown the formation of heterodimers between Robo1 and Robo4 in HUVECs [67], thus opening the possibility that slits binding to Robo1–Robo4 complexes also leads to the described effects via a Robo4-dependent, Unc5B-independent mechanism. Moreover, in vitro studies showed that Slit2 via activation of Robo1 and Robo4 inhibits pericyte migration, which might be interpreted as an additional mechanism for stabilizing endothelial integrity [51] (Fig. 11.2d). These results suggest that signal transduction of Slit/Robo4 interaction depends on Robo1 regulating vascular stabilization and thereafter influencing endothelial sprouting.
Role of Slits and Robos in Tumor Angiogenesis
A function for slits and Robos in tumor angiogenesis has also been described. In this regard, it was found that cells of several solid tumors, including malignant melanoma, rectal mucinous adenocarcinoma, breast invasive carcinoma, stomach squamous carcinoma, and hepatocellular carcinoma, express and secrete Slit2 [68]. Interestingly, Slit2 expression in human carcinoma tumor sections seemed to increase in the center of the tumor compared with the periphery [68]. This, as well as hypoxia studies in choriocarcinoma cells, leads to the assumption that Slit2 expression is dependent on oxygen levels [69]. In in vitro settings, and in contrast to other published studies, Slit2 was able to attract HUVECs and promote tube formation in a Robo1-PI3K-dependent manner [68]. Consistently, in a xenograft tumor model with human malignant melanoma cells that express Slit2, treatment with a functional blocking antibody against the first immunoglobulin (Ig) motif of Robo1 led to decreased microvessel density and tumor weight when compared with IgG control-treated mice [68]. Another cancer model of chemical-induced squamous cell carcinogenesis also linked high Slit2 expression to increased angiogenesis, in a Robo1-dependent manner [70] (Table 11.1). Similar effects have been described in the lymphatic endothelium , where Robo1 is also expressed [71]. Slit2 stimulation thereby sufficiently enhanced the migration and tube formation of lymphatic ECs in vitro. Consistently, in vivo tumor models with mice overexpressing Slit2 showed increased tumor lymphangiogenesis and lymph node metastasis [71].
Robo4, which is strongly expressed in highly active endothelium, is also regulated by hypoxia [49]. However, to date no studies have been conducted proposing a role for Robo4 in tumor angiogenesis. Thus, whether Robo4 also plays a role in tumor angiogenesis, or whether Robo4 is only required in healthy conditions and Robo1 in pathological circumstances, remains to be determined. In support of the second hypothesis, a quantitative analysis of Robo1, Robo4, and Slit2 messenger RNA (mRNA) in colorectal cancer tissue versus normal tissue revealed that while Robo1 was significantly upregulated in tumor tissue, no changes where observed for Robo4 or Slit2 [72].
Role in Pre-Eclampsia
It has also been suggested that the Slit/Robo pathway contributes to pre-eclampsia, a disease occurring during pregnancy that is characterized by high blood pressure, endothelial dysfunction , and impaired angiogenesis [69]. Analysis of slits and Robo receptors showed that Slit3, Robo1, and Robo4 are expressed in the endothelium of placental villi, and Slit2 and Robo1 are expressed in the syncytiotrophoblast [69]. In pre-eclamptic placentas, Robo1 and Robo4 are significantly increased compared with healthy controls, thus suggesting a potential pathological role for the Slit/Robo pathway in this disease [69]. It is not yet known whether there is a functional effect of the increased expression of Robo1 and Robo4.
Taken together, the available evidence suggests that Slit/Robo signaling in the vasculature possesses a stabilizing and maturating role. Further studies would need to clarify the situations where they might have an antiangiogenic or proangiogenic effect.
11.4 Ephrins and Eph Receptors
11.4.1 Introduction
Eph receptors are named after the Erythropoietin-producing human hepatocellular carcinoma cell line in which they were discovered. Eph receptors are divided into two subgroups—EphAs (EphA1–10 in vertebrates) and EphBs (EphB1–6 in vertebrates)—depending on the sequence homology of their extracellular domain [73, 74] (Fig. 11.3a). Eph receptors interact with the membrane-bound proteins termed ephrins (greek ‘ephros’ for controller), which are also divided into two subclasses—EphrinAs and EphrinBs (Fig. 11.3a). The five homologous members EphrinA1–5 are anchored in the plasma membrane by a GPI linker, and bind almost exclusively to EphA receptors [73]. In contrast, EphrinBs are transmembrane proteins possessing a C-terminal The name PDZ is derived from the first three proteins in which these domains were found: PSD-95 (a 95 kDa protein involved in signaling in the post-synaptic density), Dlg (the Drosophila discs large protein), and ZO1 (the zonula occludens 1 protein involved in maintaining epithelial cell polarity). PDZ motif, and mainly signal via EphBs [73] (Fig. 11.3a). However, reports show that members of the A and B classes interact with each other, as exemplified by EphB2 and EphrinA5 binding [75].

Fig. 11.3
Ephrins and Ephs in the vascular system. a Ephrins and their Eph receptors are membrane-bound molecules. EphrinAs interact preferentially with EphAs, and EphrinBs with EphBs. b EphB4 is primarily found on venous endothelial cells (ECs), whereas EphrinB2 expressed on arterial ECs; their signaling controls arterial-venous separation. c EphrinB2 is expressed in the angiogenic endothelium, as well as in the mural compartment, and also interacts with vascular endothelial growth factor receptor-2 (VEGFR2) and -3 in ECs. Upon EphB4 binding, EphrinB2 recruits Dab2 and Par3 effector proteins, leading to clathrin-dependent endocytosis of the EphrinB2–VEGFR complex. Thereby, VEGFR internalization serves as a crucial step for downstream VEGFR signaling, and directly affects angiogenesis. d VEGFR2 internalization is controlled by activated atypical protein kinase C (aPKC). Activation of aPKC is higher in stalk cells than in tip cells, thus leading to reduced internalization of the complex and reduced VEGF signaling in stalk cells. (Adapted with permission from Macmillan Publishers Ltd: Kitajewski [175], copyright 2011)
Eph/Ephrin signaling in neurons is extensively investigated and is involved in many contexts throughout the development of the CNS. Based on their structural characteristics, Eph/Ephrin signaling is considered a short-range neuronal wiring signal. Eph/Ephrin signaling controls the formation of topographic maps [76, 77] and, in particular, is essential in pre- and postsynaptic development, as well as synaptic plasticity [78, 79]. The molecular mechanisms through which Eph/Ephrin signaling takes place are very sophisticated and include special features such as receptor clustering and the ability to signal bidirectionally and autonomously [80]. The high degree of possible receptor/ligand combinations, as well as interactions with co-receptors, increases the signal diversity of Eph receptors and ephrins .
11.4.2 Eph Receptors and Ephrins in Vascular Development
In the field of angiogenesis, EphA2 and EphrinA1 are among the best-studied members of the Eph/EphrinA classes, even though almost all EphAs are expressed in ECs (for example, in human brain microvascular ECs) [81]. EphrinA1 is expressed at sites of developmental angiogenesis , and soluble EphA2-Fc inhibits EC migration, sprouting, survival, and corneal angiogenesis induced by VEGF [82, 83]. EphA2 knockout mice initially showed a normal vascular development [84]; however, more detailed studies have described that EphA2-deficient mice possess fewer and enlarged lung capillaries and increased numbers of endothelial sprouts, together with less pericyte coverage [85]. In addition, EphA2-deficient mice hyperreact to bacterial infection of the lung and ovalbumin sensitization by enhanced neoangiogenesis, immune cellular infiltration, and cytokine release [85]. Taken together, these investigations point to a functional role of EphA2 and EphrinA1 signaling in maintaining vascular integrity by stabilizing intracellular connection not only between ECs but also endothelial and mural cells.
EphB4 and EphrinB2 are the best-studied B-class members in angiogenesis, particularly because of their distinctive expression pattern, with higher expression of EphrinB2 in arterial ECs and EphB4 in the venous endothelium [86–88] (Fig. 11.3b). This specific expression pattern, together with the fact that EphB4/EphrinB2 function as a receptor-ligand pair able to signal bidirectionally, outlines the possibility of controlling cellular segregation and boundary formation as functional features of B-class signaling in ECs [89, 90]. An example of this is found in the zebrafish model organism, where the dorsal aorta and caudal vein derive from the same primordial structure that subsequently segregates into arterial and vein ECs. Angioblast cells migrate ventrally from this primary vessel to form the caudal vein in an EphB4/EphrinB2-dependent manner, whereas the remaining cells later form the dorsal aorta [91]. In this regard, a loss-of-function study in mice specifically targeting endothelial EphB4 pointed out that the dorsal aorta of the embryos was enlarged, whereas the formation of cardinal veins was negatively disturbed [90]. More importantly, it was observed that venous ECs were misallocated in arteries [90]. These findings highlight the prominent role of EphB4/EphrinB2 signaling for intercellular communication and tissue boundary formation (Fig. 11.3b).
The generation and evaluation of different EphrinB2 transgenic mouse lines demonstrated the essential role of EphrinB2 for proper vascular development. EphrinB2 knockout mice, or mice with ubiquitous overexpression of EphrinB2, are embryonic or neonatal lethal, respectively, due to severe vascular defects [92, 93]. The lethal phenotype of the ubiquitous EphrinB2 deletion could be rescued by crossing this line with an endothelial EphrinB2 overexpressing mouse line [92, 93], indicating that EphrinB2 expression in the endothelium is essential for survival. Analysis of a mouse line where EphrinB2 overexpression in the endothelium is temporally controlled revealed that overexpression of EphrinB2 during early development also leads to severe vascular defects and embryonic lethality. The severity of the phenotype decreases when EphrinB2 overexpression is induced in the more advanced developmental stages [88]. Consistent with these findings, the endothelial-specific knockout of EphrinB2 under the Cdh5 (vascular endothelial [VE]-cadherin) promoter showed a less-developed vascular system [88]. Therefore, these loss-of-function and gain-of-function studies are evidence that EphrinB2 is essential for vascular development in a specific temporal frame and proangiogenic manner. Interestingly, transgenic mice lacking the cytoplasmic domain of EphrinB2 also die during embryonic development due to defects in vasculogenesis and angiogenesis (similar to the EphrinB2 null mutants) [94], thus showing the EphrinB2 intracellular domain and reverse signaling is important for vascular development . The requirement of the EphrinB2 intracellular domain for developmental angiogenesis was also confirmed with a transgenic mouse line containing deletion of a single valine residue in the cytoplasmic domain (EphrinB2ΔV), which disrupts PDZ-dependent reverse signaling. EphrinB2ΔV showed impaired retina and brain vascularization with reduced vessel branching and vascular sprouts [95]. Similarly, in vitro experiments showed that EphrinB2 is a potent regulator of EC behavior by regulating the actin cytoskeleton through Rho-family small GTPases [96]. In addition, in vitro analyses show that the membrane-bound extracellular EphB4 domain is sufficient to induce EphrinB2-dependent EC invasion, proliferation, and overall survival [97] (Fig. 11.3c). While these studies clearly highlight the importance of EphrinB2 reverse signaling in developmental angiogenesis , the function of EphB4 forward signaling (upon EphrinB2 binding) in this process still remains unknown. Nevertheless, ablation of the EphB4 gene leads to embryonic lethality before E11.5 [98].
EphrinB2 is upregulated in ECs in response to angiogenic factors such as VEGF, fibroblast growth factor (FGF), hepatocyte growth factor (HGF), or activin receptor-like kinase-1 (Alk1) [a member of the transforming growth factor (TGF)-β family], whereas angiopoietin-1 (Ang1) attenuates its expression levels [87, 99]. In vivo and in vitro experiments show that EphrinB2 controls developmental angiogenesis and lymphangiogenesis by controlling EC sprouting activity rather than EC proliferation [88, 100]. EphrinB2 co-localizes and co-immunoprecipitates with VEGFR2 and VEGFR3. EphrinB2 reverse signaling (activated by EphB4) plays a crucial role in VEGFR2 and VEGFR3 receptor internalization, phosphorylation, and downstream signaling (Rac1, Akt, and Erk1/2) after VEGF stimulation [88, 95] (Fig. 11.3c). A recent study showed that a protein complex containing EphrinB2, the clathrin-associated sorting protein Dab2, and the cell polarity regulator Par3 associates with VEGFR2 in the clathrin-coated vesicle and mediates VEGFR2 trafficking towards the early endosome [101] (Fig. 11.3c, d). Atypical PKC (aPKC) acts as a negative regulator of this process by phosphorylating Dab2 and reducing VEGFR2 endocytosis [101] (Fig. 11.3d). Interestingly, sprouting ECs at the leading front of the mouse retina have lower levels of activated aPKC and higher rates of VEGFR endocytosis and turnover compared with nonsprouting ECs (Fig. 11.3d). Consistent with this model, EC-specific knockout mice for Dab2, Par3, and aPKC show vascular phenotypes that resemble those of EphrinB2 mutants [101].
Expression of EphrinB2 does not only localize to ECs but also to pericytes or SMCs [102, 103] (Fig. 11.3). EphrinB2 deficiency in the mural cell compartment led to embryonic vascular malformation and perinatal lethality [89, 103]. Analysis of the vasculature of these mice showed disrupted vessels and defects in the spatial organization of pericytes and SMCs, as revealed by microvessels showing scattered coverage with mural cells [102, 103]. In vitro, EphrinB2 expression in SMCs participated in SMC adhesion, migration, polarization, and formation of focal adhesions via a molecular mechanism involving Crk and p130(CAS) [89, 103].
In summary, EphrinB2 and EphB4 signals are essential for cellular segregation and vascular development, affecting ECs as well as mural cells in a proangiogenic fashion. Moreover, their interaction with VEGFRs, as well as their essential contribution to receptor turnover and signal transduction , makes them an integrative unit for different pathways in the concept of a neurovascular link.
11.4.3 Ephrins and Eph Receptors in Tumor Angiogenesis
Several studies report a role for EphrinB2 and EphB4 in tumor angiogenesis. In malignant brain tumors, EphrinB2 and EphB4 are expressed in both ECs and tumor cells [104]. EphB4 expressed in tumor cells leads to enhanced tumor angiogenesis via EphrinB2 reverse signaling in the endothelium [97] (Table. 11.1). Consistently, EphrinB2 functional blocking antibodies cause a reduction in angiogenesis and lymphangiogenesis in xenografted mice and a concomitant reduction in tumor growth [105]. Confirmation of the role of EphrinB2 reverse signaling in tumor angiogenesis was demonstrated using an orthotopic glioma tumor model and a skin heterotopic tumor model in the already-mentioned EprhinB2ΔV mice [95]. In these tumor models, angiogenesis and tumor growth was severely reduced compared with tumors in control mice. Moreover, similar to the studies using an EphrinB2 functional blocking antibody, tumor blood vessels in EprhinB2ΔV mice were devoid of sprouts and filopodia [95, 105]. EphrinB2 reverse signaling in tumor vessels was also shown to promote enlargement of blood vessels, the interaction between ECs and mural cells, and reduction of vessel permeability, leading to more stable tumor vessels [97, 104]. A soluble monomeric extracellular domain of EphB4 was shown to act as an antagonist of EphB4/EphrinB2 signaling and inhibited angiogenesis in vitro and in vivo as well as tumor growth in xenograft tumor models [100, 106].
With respect to other Ephrins and Eph members, few studies have involved EphA2 and its ligand EphrinA1 in tumor angiogenesis. EphrinA1 and EphA2 are expressed in tumor cells and tumor ECs [107]. Tumors grown in EphA2-deficient mice are smaller and less vascularized compared with tumors in wild-type mice [108, 109]. In addition, injection of soluble EphA2 and EphA3-Fc into tumor-bearing mice results in reduced tumor growth and reduced tumor angiogenesis [110, 111].
11.5 Semaphorins and Their Receptors
11.5.1 Introduction
Semaphorins (Sema) are membrane-bound, soluble signaling molecules that are able to signal long range via diffusion gradients, as well as short range via cell-to-cell contact. Dependent on their molecular structure, semaphorins are grouped into eight subclasses, with members of classes 3–7 expressed in vertebrates [112] (Fig. 11.4a). Semaphorins were first described in the nervous system where they function mainly as chemorepellents during axon guidance and neuronal wiring. However, similar to netrins and slits , there are reports highlighting a bifunctional role, whereby semaphorins can either repel or attract axonal growth cones, and also harbor both capacities [113]. Semaphorin signaling often induces cytoskeletal remodeling, affecting cellular properties such as shape, migration, and cellular connectivity [114]. The main receptors of semaphorins are plexins and neuropilins (Nrps). The nine members of the plexin family are divided into four subclasses (A, B, C, and D), whereas the group of neuropilins has two representative receptors, Nrp1 and Nrp2 [115] (Fig. 11.4a). Plexins are able to directly interact with membrane-bound semaphorins , whereas soluble semaphorin members (class 3 semaphorins only) often bind to neuropilins and signal through a heterodimer complex of neuropilin and plexin (with the exception of Sema3E, which binds directly to PlexinD1 and can induce signaling independent of Nrp1) [115]. The fact that neuropilins are known to be a co-receptor for other tyrosine kinase receptors (such as VEGFRs) makes semaphorin signaling an ideal pathway for studying their neurovascular properties [116].
Class 3 semaphorins are the best-studied semaphorins in the vascular system. Therefore, in this section we will focus on describing the fundamental aspects and functions of this group of molecules and their receptors in blood vessels. The emerging role of class 4 and 6 semaphorins in the vascular system will be briefly mentioned.
11.5.2 Class 3 Semaphorins in the Developing Vascular System
Class 3 Semaphorins and Plexins
Despite their main function as axon repellents in neurons, in the vascular system only Sema3s are mainly described as antiangiogenic, whereas the literature refers to members of the other subclasses primarily in an activating context. Different studies depict the different levels at which Sema/Plexin signaling regulates angiogenesis, including not only trajectory formation and vessel guidance but also interference with cellular properties such as filopodia formation or receptor presentation.
Among the different plexin receptors, PlexinD1 is highly expressed in ECs of developing blood vessels [117, 118]. Gene targeting studies in zebrafish show the importance of this receptor in vascular development . In zebrafish, a PlexinD1 loss-of-function mutation results in increased intersomitic vessel branching [119, 120]. Sema3A orthologs (Sema3a1 and Sema3a2) are expressed in the adjacent developing somites and restrict the growth of angiogenic sprouts to the intersomitic boundaries via PlexinD1 binding [118, 119]. In vitro studies show that Sema3A stimulation of HUVECs results in loss of actin stress fibers and inhibition of VEGF-induced cell migration, thus suggesting that Sema3A, expressed in the somites, repels PlexinD1-expressing intersomitic vessels and therefore guides vessels along the somite boundaries [118]. Further studies reporting on the molecular mechanisms of Sema/PlexinD1 signaling in intersomitic vessels show that Sema/PlexinD1 signaling antagonizes VEGF proangiogenic activity by controlling the levels of soluble VEGFR1 (sFlt1) expressed by ECs [119].
In a similar manner, PlexinD1 knockout mice present blood vessel patterning defects and die soon after birth [121]. Endothelial-specific PlexinD1 knockout mice (using the Tie2-Cre mouse line) consistently present defective development of the vasculature, heart, and skeleton [122]; however, Sema3A knockout mice show none or mild vascular defects, highly depending on genetic background [123, 124]. Sema3E in mice, in contrast to zebrafish , seems to be the required ligand for PlexinD1 signaling in the vascular system (independently of Nrp1). Sema3E is expressed in a caudal-rostral gradient in the developing somites, and Sema3E null mouse embryos present a similar phenotype as PlexinD1 knockout mice; however, they are viable throughout adulthood [121]. Interestingly, it was recently shown that despite severe initial defects in the dorsal aorta of Sema3E null mice (which would lead to lethality), these defects are resolved during development via morphogenetic cellular rearrangements whereby abnormal vessels remodel into normal vessels instructed by guidance cues from the lateral plate mesoderm [125]. Sema3E/PlexinD1 signaling in the vasculature has also been investigated in the developing mouse retina, where astrocyte-derived VEGF induces PlexinD1 expression in ECs of the angiogenic front (Fig. 11.4b). Even though Sema3E expression is evenly distributed in the retinal ganglion cell layer, local PlexinD1 expression at the angiogenic front restricts Sema3E signaling to the sprouting front of the retina vasculature [126, 127] (Fig. 11.4bb). Further investigation into the molecular mechanisms revealed that Sema3E/PlexinD1 signaling negatively regulates Dll4 expression in the retinal vasculature and that, in turn, Sema3E/PlexinD1 signaling is required for a balanced ratio of tip and stalk cells [127] (Fig. 11.4c).

Fig. 11.4
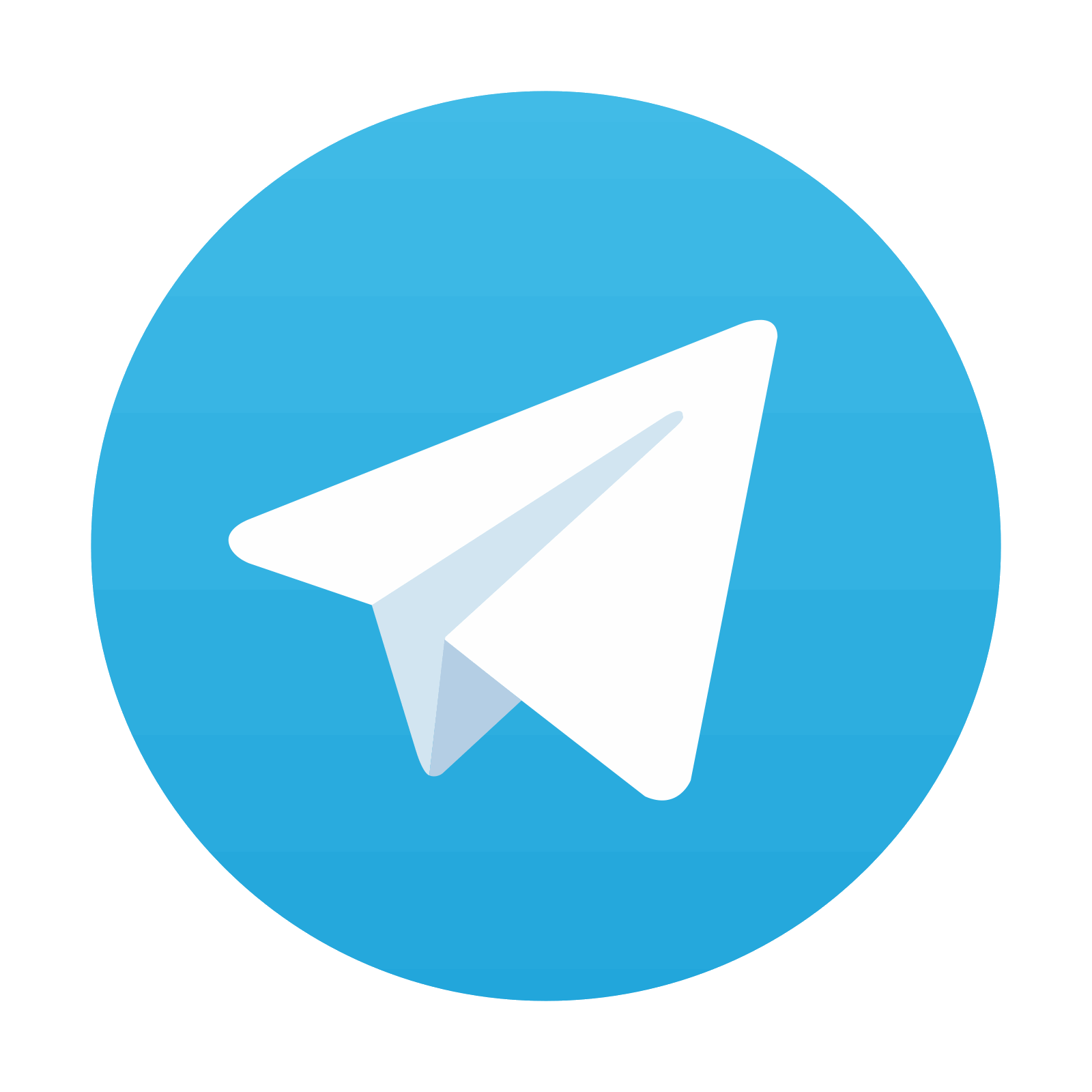
Semaphorins and their receptors. a Class 3 semaphorins are secreted molecules, whereas members of the other semaphorin classes are membrane-bound. Semaphorin and plexin receptors possess a specific Sema domain. Neuropilins also interact with semaphorins . Nrp1 and 2 are expressed in the vasculature and in smooth muscle cells (SMCs)/pericytes, whereas the expression of PlexinD1 is restricted to the endothelial tip cell (see scheme of blood vessel sprout). b In the developing retina, Sema3E is evenly expressed by retinal ganglion cells (RGC) in deeper layers. The angiogenic vasculature grows in the direction of a VEGF gradient that is generated by astrocytes, showing a higher concentration in the avascular border regions. PlexinD1 is expressed in endothelial tip cells, which restricts the Sema3E effect locally to the angiogenic front. c VEGF/VEGFR2 signaling positively controls PlexinD1 expression in the endothelial tip cell . In turn, Sema3E/PlexinD1 signaling negatively regulates Dll4 in tip cells and thus controls tip-stalk cell fate. d PlexinD1 interacts with β1-integrins and, on Sema3E binding, activates Arf6 and R-Ras, leading to internalization of the receptor complex, reduced adhesion, and filopodia retraction. e Neuropilins not only serve as a receptor for semaphorins but also bind to VEGF ligands. Nrp1 plays a crucial role in mediating VEGF signaling by interacting with VEGFR2. The presence of Nrp1 modulates VEGFR2 downstream signaling pathways (including Erk1/2, FAK, and Src). Sema3A binding to Nrp1 does not block VEGFR2 phosphorylation but inhibits its downstream signaling. (Adapted with permission from Macmillan Publishers Ltd: Kitajewski [175], copyright 2011)
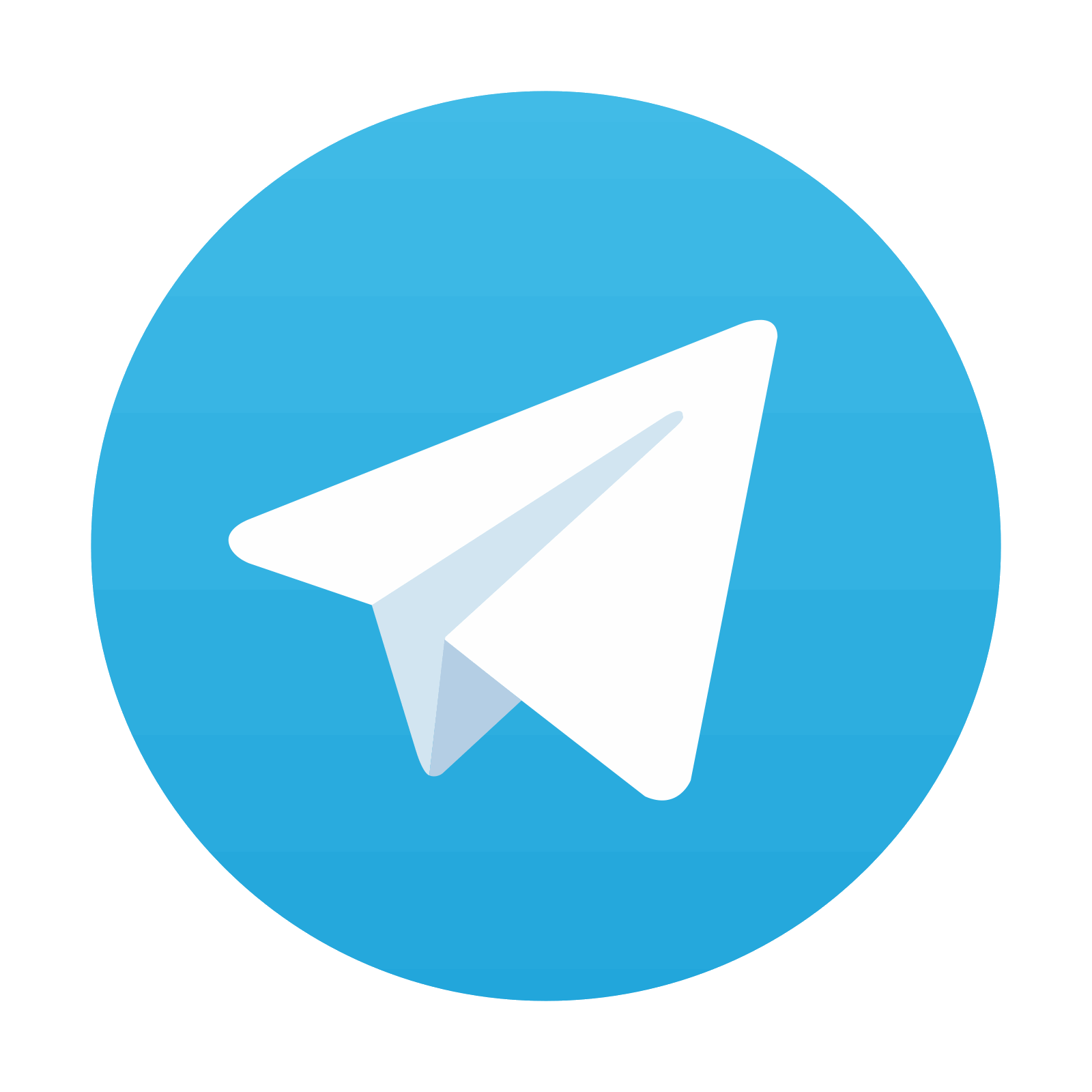
Stay updated, free articles. Join our Telegram channel
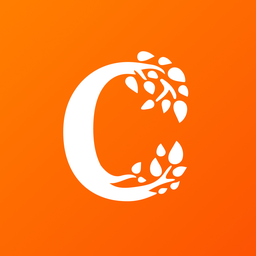
Full access? Get Clinical Tree
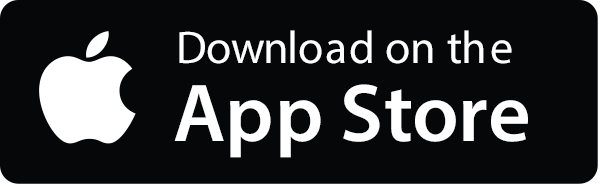
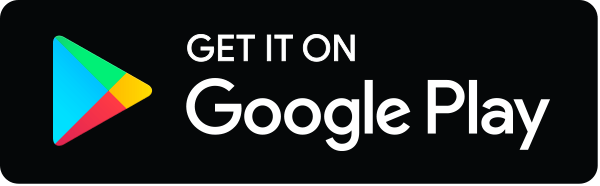