Philip T. Levy, Koert de Waal Key points Myocardial performance plays an important role in determining short- and long-term outcomes in term and preterm infants. Echocardiography is the most commonly used diagnostic modality for cardiovascular assessment in neonates; however, measuring the nature of cardiac mechanics is difficult due to the complex myocardial geometry and the interplay of cardiac loading conditions. In neonates myocardial performance can be characterized by three separate echocardiography techniques: (1) changes in cavity dimensions, (2) displacement and velocity of a single point along the myocardial wall, and (3) deformation of a segment of the wall. Until recently, the use of echocardiography in neonates to assess the adequacy of the cardiovascular system was largely dependent on either a subjective assessment of myocardial function, the use of measurements of cavity change during the cardiac cycle (e.g., shortening fraction, SF, and ejection fraction, EF), or blood flow velocity. However, not all aspects of cardiac mechanics can be identified using conventional echocardiography techniques; specifically, it is difficult to capture either the twisting motion or wall thickening for the whole ventricle. Furthermore, wall motion measurements cannot differentiate between active and passive movement of a myocardial segment. For example, on conventional echocardiography, a myocardial segment that has lost its function may still show movement due to the tethering effect of adjacent segments leading to misdiagnosis. Two emerging and validated modalities that directly assess muscle wall characteristics in neonates, tissue Doppler imaging (TDI) and two-dimensional (2D) speckle tracking echocardiography (2DSTE), enable acquisition of quantitative information that supersedes the qualitative impression provided by conventional methods.1 TDI is a modality that captures information on muscle movement velocity and cardiac cycle event timings using a high temporal resolution.2 The Doppler effect is the term given to the change in frequency of a wave reflected by an acoustic source when there is relative movement between the source and the wave transmitter and can be applied in the assessment of heart muscle (tissue) characteristics. This was first demonstrated by Isaaz et al. in their assessment of the left ventricular (LV) wall using a pulse wave (pw) frequency signal.3 TDI captures information using high frame rates (typically greater than 200 frames per second). The high temporal resolution achieved using this technique facilitates the measurement of a wide array of myocardial muscle characteristics including the velocity of muscle movement during systole and diastole, deformation measurements (also known as strain and strain rate (SR) measurements), in addition to the measurements of the timing of events within the cardiac cycle (systolic and diastolic times/isovolumic contraction and relaxation times). Those measurements can now be derived by pw tissue Doppler imaging (pwTDI) and color TDI (cTDI) (Figure 11.1). Myocardial deformation analysis is an emerging quantitative echocardiographic technique to characterize global and regional ventricular and atrial function in neonates. Cardiac strain is a measure of tissue deformation, and SR is the rate at which this deformation occurs. Myocardial strain can be measured in terms of three normal strains (longitudinal, radial strain, and circumferential) and six shear strains4 (Figure 11.2). Currently, only normal strain and shear strain in the circumferential-longitudinal plane (rotational mechanics) have been investigated for clinical use in neonates.1 These measurements can be obtained in neonates using TDI or 2DSTE.1,5,6 Characterization of cardiac performance with myocardial deformation by 2DSTE is a validated method to assess both ventricular contractility and loading conditions in term and preterm infants and provides fundamental information on myocardial properties and mechanics that would otherwise be unavailable with conventional imaging.1,5–8 2DSTE is a non-Doppler technique that applies computer software analysis of images generated by conventional ultrasound techniques. The Doppler ultrasound signal generates artifacts due to random reflections, called speckles. These speckles stay stable during the cardiac cycle and can act as natural acoustic markers. Speckle tracking software defines and follows clusters of speckles from frame to frame to calculate parameters of motion (displacement and velocity) and parameters of deformation (strain and SR).9,10 There is an expanding body of literature describing longitudinal reference ranges and maturational patterns of TDI velocity– and 2DSTE-derived strain values in term and preterm infants.1,2,10 Comprehension of principles, technical aspects, and clinical applicability of each modality is a prerequisite for its routine clinical use in neonates. This chapter will introduce the reader to TDI and 2DSTE of the LV and right ventricle (RV), as well as explore novel application of left atrial 2DSTE-derived strain, rotational mechanics, and blood speckle imaging (BSI). We discuss the expanding body of literature that details methodology (feasibility and reproducibility), terminology, and reference ranges and provides a practical guide to the acquisition and interpretation of data, and explore the diagnostic/predictive ability of all these parameters with respect to neonatal cardiopulmonary health and disease. In order to understand the relative strengths and weaknesses of all the measurements obtained using TDI and 2DSTE, a thorough understanding of the mechanics of cardiac performance is required. It is important to distinguish between intrinsic myocardial function (termed contractility) and pump function (termed myocardial performance). Contractility refers to the crosslinking of the actin and myosin filaments resulting in active myofiber force development and the shortening of sarcomeres. Myocardial performance or pump function describes the overall pressure development and deformation resulting in the ejection of blood from the ventricular cavity. Myocardial performance is therefore dependent on important physiological factors. The functional measurements obtained using TDI predominantly assess myocardial performance rather than intrinsic function and as such, interpretation of values should be done in the context of the clinical situation and loading conditions. TDI filters out high-velocity signals obtained from movement of blood to focus on the lower-velocity Doppler signals of the muscle walls. TDI can be performed in pwTDI and cTDI modes (Figure 11.1). Tissue Doppler velocities can be acquired by spectral analysis using a pw Doppler technique. Muscle tissue wall moves at a much slower velocity and a higher decibel amplitude range than blood, thus facilitating a high temporal resolution, with minimal artifact from blood.1 Recent advances have enabled the distinction between the faster-moving blood (>50 cm/s) and slower-moving muscle tissue (<25 cm/s). pwTDI assesses longitudinal velocity of a ventricular wall segment from base to apex, providing a measure of systolic function that is recorded as the peak systolic velocity of the myocardial muscle (s′ wave).11 The systolic wave is usually preceded by a short upstroke during isovolumic contraction. In addition, a measure of diastolic performance can be obtained as the ventricular wall moves away from the apex in the opposite direction. The diastolic wave is biphasic and is recorded as the peak early diastolic velocity (e′ wave) and the late diastolic peak velocity (a′ wave), which reflects the active ventricular relaxation and atrial contraction phases of diastole, respectively. The diastolic waves are usually preceded by another short upstroke during isovolumic relaxation time. The duration of the isovolumic relation and contraction phases, in addition to the systolic and diastolic times, can also be accurately obtained using this modality (Figure 11.1). pwTDI has high temporal resolution, but does not permit simultaneous analysis of multiple myocardial segments. cTDI uses phase shift analysis to capture atrioventricular annular excursions. Compared with pwTDI, cTDI increases spatial resolution and provides visualization of multiple segments of the heart from one single view. It measures mean rather than peak systolic and diastolic velocities. As a result, velocities obtained using this technique are generally 20% lower in systole and diastole compared to pwTDI imaging. The two methods are therefore not interchangeable.12 cTDI does have the advantage of combining the high temporal resolution seen with pwTDI, with a high spatial resolution. In addition to this, myocardial velocities recorded at the left and right ventricular base, and septal wall, can be obtained from a single image for later offline analysis. A comparison between left and right ventricular function can therefore be performed. Muscle tissue at the base moves at a higher velocity than that closer to the apex. cTDI can be used to assess this velocity gradient across the wall of interest (Figure 11.3). Data on cTDI values and clinical applicability in the neonatal setting are limited. This is likely due to the need for offline analysis to obtain those values and lower reproducibility when compared with pwTDI. The data presented below relate only to pwTDI. Accurate TDI velocity measurements are highly dependent on obtaining good-quality images. This may be challenging in the neonatal setting, particularly in premature infants, where lung artifact can interfere with obtaining clear images of the walls of interest. Images are most often obtained from an apical four-chamber view but can also be acquired from the apical three- and two-chamber views. The sector width of the field of view is usually narrowed to only include the wall of interest. This ensures that the temporal resolution is enhanced and a frame rate of over 200 frames per second is obtained. A pulsed wave Doppler sample is placed at the base of the LV free wall, the base of the septum, and the base of the RV free wall. The sample gate is narrowed to only capture the velocity of the area of interest (usually 1–2 mm). It is crucial to maintain an angle of insonation of <20° to prevent underestimation of velocities. This can be most challenging for the LV free wall in infants with marked ventricular enlargement due to volume overload (e.g., hsPDA). TDI velocity measurement modality will only assess muscle movement parallel to the probe beam. This is a limitation of this modality as muscle movement perpendicular to the line of interrogation will not be assessed, and as a result, TDI velocity measurements are reserved for longitudinal (base to apex) muscle tissue movement. As outlined above, TDI velocity assessment measures myocardial performance rather than intrinsic contractility thus, the values are highly influenced by loading conditions (in addition to intrinsic contractility). Increased preload increases systolic tissue Doppler velocities, while increased afterload reduces those velocities.13 Therefore clinical interpretation of those measurements must take into account the loading conditions likely to be present in the clinical situation. This has important implications for therapeutic interventions, where in some instances it may be more beneficial to improve preload (using volume support) or reduce afterload (using lusitropic medication) rather than targeting an improvement in intrinsic contractility. In addition, it is important to recognize that tissue Doppler velocity imaging cannot distinguish between active muscle movement and translational wall motion (a non-deforming segment tethered to a functioning segment). Tissue Doppler velocities may be falsely elevated, as they interrogate motion at a single point in the muscle wall with reference to the ultrasound transducer,2 whereas deformation imaging (see later) easily differentiates the two. The use of TDI has expanded in recent years as assessment is highly feasible in small infants and has been validated in term and premature neonatal populations14,15 as well as in fetuses.13 Reference values for term infants are shown in Table 11.1.16–18 Several studies have documented serial changes in those functional measurements over the first postnatal day and up to 1 year of age.16–20 In term infants TDI can be used to assess RV function to provide important clinical prognostic information in infants with congenital diaphragmatic hernia21 and may be used to monitor treatment response in infants with acute pulmonary hypertension (aPH) of the newborn.22 The superior sensitivity of TDI to subtle myocardial dysfunction when compared with shortening or ejection fraction in term infants was recently demonstrated. In infants born to mothers with diabetes mellitus (of any cause), left and right ventricular systolic function measured using TDI velocity is lower than control term infants. This occurs without differences in shortening fraction between the two groups.23 Providing reference ranges for preterm infants is more complex.2 Tissue Doppler velocity imaging is dependent on the gestational age with lower myocardial velocities in both systole and diastole at lower gestations.17,19,24 Furthermore, many preterm infants in the studies had conditions and treatments that influence preload and/or afterload, such as mechanical ventilation, PPHN, or a PDA. In premature infants pwTDI velocities have been used to predict clinical deterioration following patent ductus arteriosus (PDA) ligation and as a guide to institute targeted therapy. In addition, they can be used to assess treatment response in this scenario when conventional measures of function, including shortening and ejection fraction, are not sensitive enough.25 Incorporating TDI for the assessment of myocardial performance in the setting of a PDA during the first few days of age may facilitate a more targeted approach to PDA treatment25 and facilitate the development of prediction markers for adverse outcomes. Of note is that there is also an emerging association between lower diastolic function measured using TDI and chronic lung disease in premature infants.25 Myocardial deformation refers to the change in shape of the myocardium in several planes, from its baseline shape in diastole to its deformed shape in systole. This occurs as a consequence of myofibril shortening. As the myocyte unit is not compressible, deformation of the ventricles occurs while maintaining the overall muscle volume resulting in a reduction of the ventricular cavity volume. Any shortening in one direction leads to expansion in other directions. The developing heart starts out as an isotropic tissue built of cardiomyocytes and supportive tissues and gradually develops into an anisotropic tissue where groups of cardiomyocytes are structured as laminar sheets of fibers.26 In the subendocardial region the fibers are longitudinally oriented along the axis of the heart with an angle to the right (right-handed helix); in the midwall the fibers are circumferentially orientated; and in the subepicardial region they are longitudinal again but with an angle to the left (left-handed helix)3 (Figure 11.4). This double helical structure of the heart is important for its function. Being able to shorten in longitudinal and circumferential directions simultaneously, thus creating a twisting motion greatly improves energy efficiency and the ability to empty and fill the heart with blood. Myocardial motion thus consists of narrowing, shortening, lengthening, widening, and twisting. As mentioned, myocardial strain can be measured in terms of “normal” strain and “shear” strain.4 Normal strain is caused by forces that act perpendicular to the surface of the myocardial wall, resulting in stretching or contraction without skewing of the volume.27 There are three types of normal strain: longitudinal, radial, and circumferential. Conversely, forces causing shear strain act parallel to the surface of the wall and lead to a shift of volume borders relative to one another as delineated by a “shear” angle.27 There are six forms of shear strain grouped into three categories: circumferential-longitudinal, circumferential-radial, and longitudinal-radial. Myocardial shear in the circumferential-longitudinal plane results in twist or torsional deformation of the LV during ejection. Only the three normal forms of strain and circumferential-longitudinal shear strain (rotational mechanics) have been investigated for clinical use in neonates.1 LV and RV deformation patterns differ based on their own unique myoarchitectural fiber orientation. The LV myocardium consists of circumferential fibers in the midwall layer and longitudinal fibers in the endocardial and epicardial layers.28 The left ventricle (LV) deforms in three planes, including longitudinal (base to apex), radial, and circumferential. The LV shortens longitudinally and circumferentially but thickens in the radial plane. This facilitates the maintenance of muscle volume while reducing the volume of the ventricular cavity to facilitate the election of blood during systole. During diastole, the ventricle returns to its baseline un-deformed shape (Figure 11.5). In the circumferential-longitudinal plane the net difference in the systolic rotation of the myocardium between the apical and basal short-axis plane is referred to as twist (degrees) and represents the wringing motion of the LV during systole. If normalized to the distance between the respective image planes, it is referred to as torsion (degrees/cm). LV rotational mechanics (twist and torsion) are assessed by STE. Compared with the LV, the RV myofiber architecture is composed of superficial oblique and dominant deep longitudinal layers. The myofibers in the RV are aligned in a more longitudinal direction than in the LV, and as the dominant pattern of RV deformation, longitudinal shortening provides the major contribution to stroke volume during systole and is a more sensitive indicator of RV dysfunction.4,8,29 Deformation in the circumferential and radial directions in the RV may prove to be a valuable measure of function in certain neonatal conditions (i.e., congenital heart disease), but there is a paucity of studies that use these measures in clinical practice and those studies have not been able to demonstrate significant reliability in neonates.30,31 Deformation imaging has the advantage over velocity imaging in distinguishing between movement due to tethering and true deformation. Velocity imaging will register a displacement velocity of this non-deforming segment as it may be tethered by an adjacent functioning segment. However, an infarcted segment of the myocardium will not deform and its strain values will be close to zero. Strain can be used to assess regional and global myocardial performance. Strain is analogous to ejection fraction and as a result, it will be dependent on loading conditions as well as intrinsic contractility. SR on the other hand is thought to be less dependent on preload and afterload, and as a result, it may represent a more accurate reflection of intrinsic contractile function. Deformation imaging may therefore be used to distinguish between reduced performance resulting from loading conditions (which will affect strain but not SR) and reduced performance resulting from intrinsic contractile dysfunction (which will affect both strain and SR).32 There are two established methods for assessing and calculating deformation entitled Lagrangian strain and Eulerian (natural) strain.33 Lagrangian strain refers to the change in length relative to an unstressed baseline length, against which all subsequent deformation will be measured.34 Since Lagrangian strain is measured as the separation distance between two regions of myocardium relative to the original separation distance in end-diastole, it is not affected by the heart rate.35 STE lends itself more readily to the calculation of Lagrangian strain, since the baseline length is always known and can easily be used as a reference. Eulerian strain calculation is based on a reference length that is different at each interrogation time point, for example, each color tissue Doppler frame, and is better suited for use with TDI. Natural and Lagrangian strains are related so that one can be converted into the other. STE software packages will report Lagrangian strain, but natural strain (i.e., tissue Doppler) can be derived from STE by conversion from the Lagrangian strain. Studies that utilized strain and SR measures to characterize function in neonates must therefore indicate the software package and the type of strain or SR. TDI-derived strain is not discussed in this chapter and is referenced elsewhere.1,2 Strain (S) is the percent change from its original length, with negative values describing narrowing or shortening and positive values lengthening or thickening. SR is the average change in strain per unit time and is expressed as 1/s. Peak systolic strain occurs at the end of systole at aortic valve closure. Negative strain is used to express shortening (in the longitudinal and circumferential planes) and positive strain is used to express radial thickening. The nomenclature is longitudinal for base-to-apex, circumferential for rotational, and radial or transverse for inward motion and deformation. The difference in the rotation of the myocardium between the apical and basal short-axis plane is commonly referred to as twist and reported in degrees. If rotation is normalized to the distance between the respective image planes, it is referred to as torsion and reported in degrees/cm. Normalizing twist to LV length facilitates comparison of LV rotational mechanics across differing age groups, but this can only be done accurately with 3D imaging. Recommended nomenclature, units, and abbreviations for 2DSTE-derived parameters are provided in an important recent consensus report.8 The report also provides consensus on timing of mechanical events, essential for standardization of almost all derived parameters of motion and deformation. To be able to report on Lagrangian strain (where the original length is known), the software would need a reference point in time (the beginning of the cardiac cycle) and an endpoint where deformation is determined according to its reference point. End-diastole is commonly taken as beginning of the cardiac cycle, either determined from the four-chamber images as the frame before the mitral valve completely closes or using surrogates such as the R peak of the ECG or the largest volume of the LV. End-systole is the usual endpoint and coincides with aortic valve closure, determined from apical long-axis views or using surrogates such as the end of the T wave of the ECG, the end of the negative spike after ejection on the 2DSTE-derived velocity trace, or the minimum volume of the LV.9,10 The current consensus in adult cardiology is to report on end-systolic strain, with other parameters reported in addition (Figure 11.6). Most papers on neonates report on peak systolic strain (the first peak S value found) or maximum systolic strain (the highest S value found). Post-systolic strain has not been systematically reported in neonates but could prove to be an important parameter in fetal growth restriction.36 As the heart contains layers of fibers in different directions, motion and deformation can vary depending on where in the myocardial wall it is measured. (i.e., at the endocardium, midwall, or averaged over the entire cardiac wall).37 Whether this also plays a role in the analysis of the thin-walled and immature neonatal myocardium is unclear. Older software versions generally report on endocardial deformation, while the newer versions report on (the lower) global or midwall deformation, with separate reporting for each wall area. To help interpret the data, it is important to understand the difference between global and segmental motion and deformation. The term global deformation should be reserved for the combined findings from the four-chamber, two-chamber, and long-axis apical views, or from the basal level of the papillary muscle and apical short-axis views. Segmental deformation can be vendor specific, as the segmental model used could describe 16, 17, or 18 segments.38 Most software packages would divide the ventricular wall into six equidistant segments termed basal, mid, and apical segments for the apical views and anatomically for the short axis views (e.g., anteroseptal, anterior, lateral, posterior, inferior, and septal wall segment). Data can be reported as global (all segments of all views), four-chamber view alone (six segments), per ventricular wall (three segments of the LV free wall, RV free wall, and the septum, respectively), or per individual segments (i.e., basal segments only for 2DSTE-derived myocardial velocities). Most 2DSTE data in neonates have been determined from the apical four-chamber view alone, as not all software can calculate global parameters when the heart rate varied too much between views. Speckle tracking analysis can be performed on normal 2D grayscale images but does require some adjustments to optimize feasibility of successful analysis. As mentioned earlier, 2DSTE tracks artifacts that are there because of random reflections of the Doppler signal; hence the optimal image should contain a combination of a perfect 2D grayscale image with enough artifacts (speckles) within the myocardial wall. This is not always easy to achieve. The feasibility of 2DSTE analysis in neonates has been reported to be between 70 and 95% of the images acquired, with the highest feasibility in the more recent studies.1 As with any ultrasound imaging, the poorer the image quality, the less useful the analysis. Images selected for analysis should be reviewed for image quality before analysis is attempted. Using a subjective or objective image quality scoring sheet can assist in increasing the feasibility of 2DSTE analysis.39 Quality items include completeness of the ventricle, how the endo- and epicardial borders appear, the presence of artifacts, the ECG trace, and frame rate used (Table 11.2). For optimal clarity of the endocardial borders, the overall gain setting and time-gain compensation should be adjusted to produce images that appear brighter than that typically used in conventional echocardiography. Neonates are often not sedated for image acquisition, so motion along with breathing can cause some segments to move in and out of the plane of view.
Chapter 11: Advanced cardiac imaging in the newborn: Tissue doppler imaging and speckle tracking echocardiography
Introduction
Principles of cardiac function
Tissue doppler velocity imaging
Pulsed wave tissue doppler velocity measurements
Color tissue doppler velocity
Measurement of TDI velocities
Clinical application of TDI velocity measurements
Day 1
Days 2–3
Day 28
s′ (cm/s)
LV free wall
5.0 (3.5–7.0)
5.0 (3.5–7.0)
6.0 (5.0–8.0)
Septum
4.5 (3.0–8.0)
4.5 (3.0–8.0)
RV free wall
7.0 (5.5–9.0)
7.0 (5.5–9.0)
9.7 (6.0–12.0)
e′ (cm/s)
LV free wall
6.5 (5.0–8.5)
6.8 (5.0–9.0)
8.6 (6.0–11.0)
Septum
5.0 (3.8–6.0)
5.0 (4.0–7.0)
RV free wall
8.5 (6.0–11.0)
8.0 (6.0–11.0)
10.5 (6.0–14.0)
a′ (cm/s)
LV free wall
6.5 (4.5–10.0)
5.5 (4.5–9.0)
8.2 (6.0–11.0)
Septum
4.5 (3.5–6.0)
5.0 (4.0–8.0)
RV free wall
9.0 (7.0–12.0)
8.5 (6.5–11.0)
14.5 (6.0–18.0)
Ee′ ratio
LV free wall
8.5 (6.0–10.0)
8.1 (6.0–9.8)
7.5 (6.0–10.0)
Septum
9.2 (7.0–11.0)
9.5 (7.0–11.0)
RV free wall
5.8 (5.0–8.0)
6.0 (5.0–8.0)
6.0 (5.0–11.0)
IVRT (ms)
54 (40–70)
53 (40–70)
42 (35–50)
Derived deformation measurements
Principles of deformation imaging
Basic concepts and terminology of deformation
Image acquisition
Advanced cardiac imaging in the newborn: Tissue doppler imaging and speckle tracking echocardiography
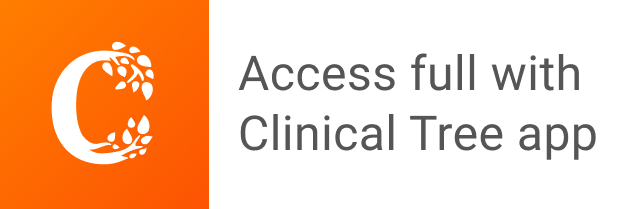